Automation: what will the cell therapy laboratory of the future look like?
Cell Gene Therapy Insights 2018; 4(9), 679-694.
10.18609/cgti.2018.067
With the recent successes of CAR-T cell therapies there has been a renewed interest in bioprocessing and the manufacturing facilities required to meet the demands. Increasing the scale of any process has potential risks as well as rewards. While the drive to automation is clear, there is a lot of debate on the strategy. The decision on whether and when to automate a particular step can have long-term consequences on the therapies’ cost-of-goods (COGs) and their successes. Automating the most complex and error-prone steps of a process is thought to be the best alternative to manual processes. However, automation may not be possible or even recommended for all steps in a process. Here, we examine both the barriers and the advantages of automation. Additionally, a detailed discussion of automating the autologous CAR-T cell process will look at each step from collection to infusion, and discuss the current automation offerings and speculate on potential future process changes. Through application of process analytics, we can gain a deeper understanding of the processes’ consistency and tailor automation to fit the process. New potency assays will improve manufacturing processes and ensure the delivery of a safe and effective product. These advancements will then shape the cell therapy laboratory of our future.
What will the bioprocess manufacturing facilities of the future look like? To answer this question, we must firstly envision cell-based products as a mainstream therapeutic option – this then calls for the therapies to be both affordable and accessible. These two concepts will guide the strategy and therefore design of the manufacturing facility.
Firstly, the product must be affordable for public acceptance as a therapeutic option. With the recent FDA approval of CAR-T cell therapy Kymriah® (CTL019) from Novartis, and Yescarta® from Kite Pharma (a Gilead company), there has been much debate on what the cost of therapies should be. Unfortunately, there is a large discrepancy between the expected and actual selling price of a cell therapy [1]. The International Society of Cell Therapy (ISCT) subcommittee posted survey results that showed labor and materials to be an estimated average of 61% of the total COGs. In terms of constraints in manufacturing that were ‘rate limiting’ and contribute substantively to increased costs, respondents cited ‘lack of automation’ and ‘manpower’ ahead of regulatory concerns, materials, and testing [2].
Secondly, the product must be accessible to patients. An informed decision on manufacturing strategy – centralized versus decentralized, scale-up versus scale-out – will need to take into account their benefits and risks. For example, one of the biggest considerations for centralized manufacturing is cold chain logistics. Careful management of this process is required in order for the cellular product to maintain viability and functionality. Most survey respondents (55%) will utilize liquid nitrogen storage for their products in development and more than half will ship products in frozen form, and cold chain logistics were estimated to be averaged 7% of COGs; this significant cost thereby underscores the need for alternative, cheaper technologies [1]. On the other hand, some models will utilize decentralized manufacturing facilities which will reduce the need for cold chain logistics, but will place importance on product and process consistency between different sites. Then, the ease of process transfer becomes critical.
It has been suggested that the COGs for a particular process are essentially defined and locked in with the investigational new drug (IND) application [3]. It is therefore imperative to design the pre-clinical research with lean manufacturing in mind, to reduce the COGs at the commercial stage. The concept of lean manufacturing was introduced through the manufacturing success of Toyota [4] and has since been utilized in many industries [5–7]. The overarching idea is to scrutinize every activity within the manufacturing process and then eliminate any wasteful or non-value adding activities to achieve optimal use of facility space, labor and equipment [4]. Some examples of waste are: unnecessary motion due to poor facility design; underutilization of talent by wasting skilled labor resources on simple, manual processes; waste of materials and labor on defective products; overproduction that results in excess stock inventory; and performing processing and tests not necessarily required by the end customer [4]. Further discussion is outside the scope of this article, but the opinion of the authors is this: automating and closing the most complex and error-prone processes can possibly reduce much of this waste. Furthermore, the drive towards automation can clearly help enable process scalability and ease of transfer, but there is still debate on when automation should be adopted [8].
Here we will examine some of the barriers to automation as well as the potential advantages. Possible automation solutions for the various steps in the cell therapy manufacturing process of a CAR-T product will be examined. Current process solutions will be presented and potential solutions for the bioprocess lab of the future will be discussed.
Barriers to automation
The Catch 22 of cell process development for cell therapy is that to automate you need to have a process that can be automated. This usually requires a well understood process and a strategy for making the desired changes. In some cases, the first role of automation is to provide data to increase process understanding. As understanding increases, so should the ability to optimize and control a process. For example, cells destined for a clinical trial should be a consistent and uniform population. However, the cell expansion process inherently produces heterogeneity within the batch, most likely due to small differences in the cell microenvironment. Human factors are the largest contributor to these differences so automated solutions that reduce heterogeneity will by default improve product quality.
Current challenges to cell therapy manufacturing can be grouped into four areas: quality, scalability, sustainability and robustness of the supply chain and COGs [9].
Several aspects of cell therapy manufacturing will have an impact on product quality and COGs. Patient-specific cell therapies are much different than “off the shelf” therapies such as traditional biologics. Compared with processes for traditional biologics, autologous cell therapy manufacturing is very manual and labor intensive. By one estimate, there are three-times more hands-on operations than biologics manufacturing. Extrapolating from a current biologics manufacturing batch failure rate of 3%, one would expect at a minimum a 10% failure rate in cell therapy processes [10]. Such complex processes can be difficult to automate and optimize because when the “process is the product”, changes in the process can lead to comparability issues [11]. When switching from a manual platform to a single-use automated platform, care should be taken that the disposable bags and tubing sets do not introduce leachable substances or particulates to the product [12]. Leachable substances and extractables are not restricted to single-use disposables and can be present in almost every unit operation of a biomanufacturing process affecting product quality, stability, consistency and even patient safety [13,14].
Failure of a lot can be life threatening to the patient waiting for treatment which creates regulatory demands of regenerative medicine materials that are much greater than for molecular pharmaceuticals and much more rigorous than for most industries [15], consequently driving up the COGs. Regulatory requirements call for a separate batch record and lot release for each patient and parallel processing of multiple patient lots simultaneously, thereby limiting economies of scale.
There are many reasons to delay automation of a cell therapy process including the lack of suitable devices. Each process for the manufacture of CAR-T cells is unique. Current hardware solutions offer poor compatibility especially when it comes to single-use disposables. Likewise, a software platform capable of interacting and controlling all the devices in a process does not exist [16]. These complex processes will require new hardware designs to match the unit operations. Custom hardware will be costly and may require change as process understanding increases [17].
An outsourced automation solution will be quicker and less expensive, however greater control and flexibility can be maintained with an in-house automation solution, be it modular or end-to-end [16]. There are two main sources of error in any bioprocess: human error and the unexpected outcomes due to a lack of process understanding [18], both of which can be more efficiently managed using an in-house automation solution.
The challenge of automation is how much of the process can be standardized and which parts need to be customized. This includes not just the available hardware, but also there must be a plan to deal with the patient. Most processes are developed with healthy donors. Later, when the researcher has access to patient material the process can be standardized to meet critical quality attributes. Obviously, keeping most of the process standardized and using custom procedures only when needed is one way to control costs [15].
Although not necessarily a barrier to automation, the idea of decentralized manufacturing would set the bar for automation quite high. To ensure comparability of product among multiple sites would require a completely automated manufacturing process and automated test methods. In fact, the comparability of manufacturing protocols needs to include collection protocols and transport between the manufacturing site and hospital [19].
Regardless of one’s overall strategy for commercialization, COGs will be both a driver and a barrier of automation. Increased reliability, consistency and reduced manufacturing costs are on the plus side of the equation and capital expense, lack of suitable equipment and lack of experience are on the minus side [3].
Advantages of automation
Automation can reduce or eliminate the number of open operations to mitigate the risk of microbial contamination. For cell therapy processes, this is particularly important as the final product/cells cannot be filter sterilized [17]. Microbial contamination and other product non-compliance also contributes to COGs when the cell product is lost. Each manufacturing unit must be capable of processing each individual patient’s cells [20]. The ability of automated systems to sense the culture conditions and respond to the different condition of each patient’s cell health can greatly benefit autologous therapies.
One of the first steps of any automation campaign should be to define the lot size and the desired characteristics of the final product. Next, automation of a manual process requires defining the individual unit operations. This will drive the equipment usage, scale of automation and quality control testing that will be required [17]. Process mapping to create a process flow diagram plus information from real time process monitoring (process analytical technologies: PAT), as well as an understanding of critical quality attributes can reduce the risk of comparability problems with the automated process [17]. Process mapping and knowledge gained from PAT can define which existing equipment can meet a users’ needs rather than allowing the equipment to define the process. Finally, an automation campaign should be stage dependent and plans must be flexible to change as the product moves toward the clinic.
Decentralized manufacturing (as mentioned above) is supported by the fact that less skilled workers can perform automated processes, allowing workers to be at different manufacturing sites and yet perform the same process.
The risk of scale-out of autologous processes is somewhat ameliorated by physically connecting and automating these complex manufacturing steps [21]. Two examples of the all-in-one solution for autologous manufacturing include the CliniMACS Prodigy® (Miltenyi Biotec) and the Cocoon™ (Octane/Lonza), which are described in a later section.
Although automation is usually considered a financial drain, calculated payback timelines for automation versus manual processing are eight to 25 months with the greatest benefit being seen when patient pools reach 1000 patients [22]. In fact, a partially automated process is more flexible and cheaper than a fully automated process especially when increased production is required [23].
Automating the autologous CAR-T cell manufacturing process
To automate a multistep process like the processing of a CAR-T product, it must first be broken down into key unit operations (Figure 1). The granularity of these key unit operations may be limited by the bioprocess equipment available for each step of the process. It also may reflect the current understanding of the process and likely be phased as process knowledge increases.
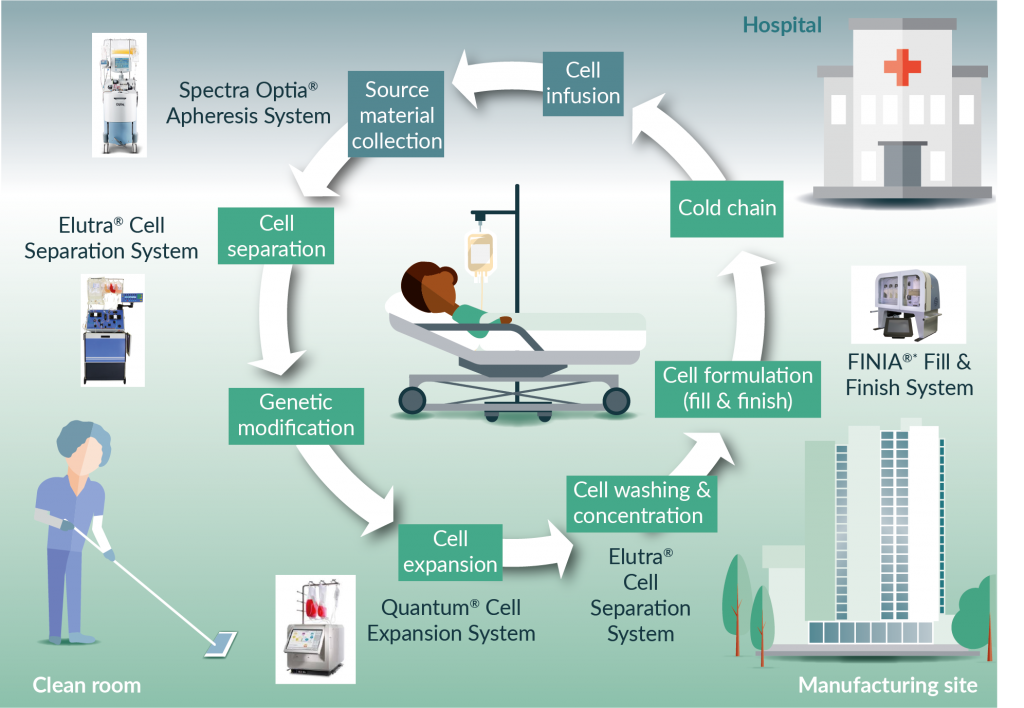
Figure 1. Automating the autologous CAR-T cell manufacturing process. * Under development TerumoBCT.
In broad terms there are four types of automation [11]:
- Process automation such as a closed loop cell expansion bioreactor;
- Task automation such as cell washing and formulation;
- Test automation which seeks to automate the testing of critical process parameters such as potency; and finally
- Whole factory automation which takes the idea of a unit operation to a whole new level with manufacturing execution systems and electronic batch records.Automation management should also include data collection through a laboratory information management system (LIMS). This allows for electronic batch and lot-release records as well as cell tracking from source material collection through transfer to a cell processing site(s), and finally re-infusion in the patient [17].
Collect source material
Most autologous cell therapies are developed with samples from healthy donors that are available in the research laboratory setting. When considering clinical applications, it becomes clear that patient samples likely need to be collected at a site (hospital) distant from the actual manufacturing site. Transportation to the manufacturing site also needs to be optimized and standardized. Sample transportation must include a standardized sample tracking process to satisfy all applicable regulatory documentation requirements.
Many CAR-T manufacturing protocols begin with the collection of peripheral blood mononuclear cell (PBMC) concentrates. However, the apheresis product may contain varying numbers of red blood cells (RBCs), monocytes, neutrophils, platelets, blast cells and myeloid-derived suppressor cells in addition to lymphocytes. These other cell types can play a determining role in the success of the CAR-T manufacturing process [24]. It has been demonstrated that controlled consistent collections enable the successful manufacturing of regenerative medicine products [25]. In fact, depletion of monocytes and granulocytes from PBMC concentrates improved the expansion of CAR-T cells [26]. The apheresis product may be collected by one of several devices, such as the Fenwal Amicus Separator (Fresenius Kabi), or the Spectra Optia® Apheresis System (TerumoBCT). If multiple device types are used, increased variability may result and may be compounded by patient to patient variability. The Spectra Optia is used to produce a high percentage of cell collections in the world today.
Once the patient’s cells have been collected, the apheresis product and accompanying documentation must be transported to the manufacturing center. Regardless of whether the cells are to be transported fresh or frozen, their journey must be documented. If the cells are frozen, they require a cold chain that can continuously monitor and document shipping conditions.
Not all automation carries risk for example: switching from manual to electronic record keeping would carry little risk [11]. Radio frequency identification (RFID) tagging can be used to connect ‘islands of automation’. This is key to autologous manufacturing schemes but can also be part of an electronic handshake used to monitor temperature controlled conditions or other processing requirements [15]. Obviously, central or dispersed manufacturing is a major business decision. If the cells must be frozen for transport then it will be imperative to build process learning around the performance of frozen versus fresh cells.
Cell separation/enrichment
A protocol may call for further fractionation of the cells when they reach the manufacturing site. Cell types can be separated according to size either using centrifugation alone or by a density gradient medium, such as Ficoll® to eliminate unwanted blood cells and isolate a ‘buffy coat’. The buffy coat represents less than 1% of the total blood volume and contains most of the white blood cells and platelets.
The COBE® 2991 Cell Processor (TerumoBCT) has been used by blood banks to deglycerolize frozen cells and can play a similar role for washing cells to remove the anticoagulant containing leukapheresis buffer in a centralized manufacturing facility. The Elutra® Cell Separation System (TerumoBCT) is designed for automated closed cell separation when sorting cells by size and density. Similarly, the Sepax 2 Cell Separation System (GE Healthcare) may be used to enrich cell types from apheresis products. Many of the tools of the blood banking industry have found purpose in cell therapy manufacturing [12]. Automation of cell fractionation is not capable of eliminating patient cell variability, but automation can reduce compounding variability resulting from human factors to potentially provide a better product with more consistent results and a higher purity of starting material [20].
Specific cell types may be separated based on cell surface markers. These separations rely on antibodies for specificity. The antibodies are coupled to fluorescent markers or magnetic beads. Both positive and negative selections may be performed using antibody tagged beads or fluorochromes. The aim of negative selection protocols is the depletion of all cells except the desired target cells in this case CD3+ T cells. Positive selection, on the other hand selects only those cells containing the desired surface marker. Negative selection is leakier but is still useful with kits reaching 95% efficiency versus 99% efficiency for positive selection kits [27]. Care must be taken to include markers for all of the unwanted cells in a negative selection cocktail.
Fluorescence activated cell sorting (FACS) is routinely used in reseach environments to select specific cell populations based on surface markers. FACS is inefficient at sorting large populations of cells because throughput is slow and the droplet-based separations are susceptible to contamination. Closed cartridge based FACS systems have been introduced by Nanocollect (WOLF Cell Sorter) and Miltenyi Biotec (MACSsQuant® Tyto®) to alleviate this problem but sorting rates remain relatively slow at 30,000 events per second.
Magnetic-activated cell sorting utilizing antibodies conjugated to magnetic beads is currently the most common method for the isolation of cell sub-populations and is quicker and more easily scaled than FACS. Additionally, closed systems for magnetic bead separations have been developed by Miltenyi Biotec (CliniMACS Prodigy) and ThermoFisher (CTS Dynamag). One disadvantage of these systems is that the magnetic beads must be removed prior to treatment, adding cost to the overall process.
Novel technologies for cell separations using microbubbles and sonic waves are under development and have shown promising results [28]. Expected improvements in antibody-based separations will increase sensitivity and reduce both cost and processing time. Increased understanding of the target cells will reduce the number required for treatment and could also make – as yet – unknown purification techniques possible.
Activation
There are a number of methods currently used to activate T lymphocytes including cell-, bead- and antibody-based activation. While a more ‘in-vivo’ like stimulation may be attained using a patient’s own antigen presenting cells (APCs) to activate T cells, there are several potential drawbacks. The time and cost of purifying these cells, patient-to-patient variability, and the difficulty of their eventual removal from the final cell mixture represent substantial challenges to the activation protocol. For some cell types such as natural killer (NK) cells, artificial APCs genetically engineered to express specific antigens have been effective [29].
The most common methods for activating T cells are bead based, such as the CD3/CD28 magnetic Invitrogen Dynabeads (ThermoFisherScientific) [9] which represent a class of reagents that have limited availability for commercial processes due to exclusivity agreements. A non-magnetic bead-based product, MACS GMP Cell TransAct (Miltenyi Biotec), consists of CD3/CD28 agonists attached to a polymeric nanomatrix; excess reagent can be removed from the cells by centrifugation. This circumvents the magnetic de-beading step required with Dynabeads. Immunocult T Cell Activator (STEMCELL Technologies) consists of cross-linked CD3/ CD28/CD2 antibodies attached to a linker domain and can also be removed by centrifugation but is, as of this writing, only available at research grade. Recently, Quad Technologies (now Bio-Techne) has developed a T cell activation product that does not rely on magnetic beads but, rather utilizes a biocompatible and dissolvable polymer technology named QuickGel™ which can be customized to present various costimulatory ligands.
Automated activation processes can improve the homogenous mixing and contact between the activation agent of choice and T cells. While this is normally performed manually, as the number of patients being treated increases it will become necessary to automate this task. The use of beads to activate cells brings up the issue of building a robust supply chain while also managing the COGs.
One of the first challenges of a cell therapy manufacturing process is to find high quality ingredients that meet good manufacturing practice (GMP) standards. Because the cell therapy manufacturing field is in its infancy, GMP quality reagents are not always available and raw materials of biological origin are sometimes required [30]. As the industry grows, increasing pressure will be placed on the providers of reagents. For example, demand for fetal bovine serum (FBS) could eventually exceed supply [11] not to mention the possibility of a regulatory change requiring FBS to be removed from all clinical products.
Supply chain management is a major business risk and as such needs to be considered early in process development. Early attention to the reagents used in a process can help control process costs. Minimizing or eliminating the use of expensive beads, kits and other proprietary reagents already in your baseline manual process will support keeping costs low.
Genetic modification
There are many methods to put the CAR in CAR-T cells. Viral vectors are commonly used to introduce the chimeric antigen receptor constructs to T cells. Retroviruses, lentiviruses, and adenoviruses are commonly used to deliver genetic modification agents to cells. Each virus has a unique packaging capacity and the ability to deliver RNA or DNA to dividing or non-dividing cells. Transposons such as Sleeping Beauty, piggyBac, zinc finger nucleases (ZFNs), transcription activator-like effector nucleases (TALENS), and a host of transfection reagents are also used to genetically modify cells. CRISPR/Cas9 technologies have shown promise in the ability to knock out and knock in genetic constructs by adding and removing specified sequences. Each of these methods carries the risk of unintended mutagenesis depending upon the degree of specificity of integration in the host genome. CRISPR/Cas9 technologies have a high degree of integration specificity as the genome editing properties are directed by a guide RNA that can be adapted to modify a desired site in the host genome [31].
In addition to viral vectors numerous chemical, electrical and even mechanical methods have been used to introduce genetic modifications. Nucleofection, lipofection, calcium phosphate, and polyethylenimine transfection reagents form complexes with the DNA and promote uptake by the cells. Electroporation applies an electrical pulse to the cells that allows the DNA and RNA to pass through small, transiently induced pores in the membrane [32]. Recently, a novel technology called Cell Squeeze® (SQZBiotech®) which utilizes a rapid mechanical disruption of the cell membrane that allows target molecules to pass without the use of chemical agents has shown efficacy in delivering molecules including siRNA, quantum dots and carbon nanotubes [33]. Transient transfection of messenger RNA by electroporation has shown utility in the laboratory to quickly check new constructs.
While it is beyond the scope of this article to discuss all the methods of genetic modification, it is important to automate this task. Automation will serve to increase the consistency of cell transfection by maximizing cell contact with the viruses and other enzymes. Several companies have automated electroporation technologies to allow for reproducible and scalable genetic modification of many cell types. MaxCyte® Scalable Transfection Systems (MaxCyte) uses an automated low-volume electroporation device that incrementally processes up to more than a liter of cells and can introduce plasmids up to 14 kB in size under current GMP guidelines [34]. Miltenyi Biotec has developed an add-on platform – the CliniMACS Electroporator – which allows for the use of automated electroporation in a GMP-compliant system and can be used with the CliniMACS Prodigy (see below) to produce cellular products for regenerative medicine applications [35].
In the lab of the future a small number of relevant cells could be specifically modified using CRISPR/Cas9 with a custom guide RNA introduced into the cells using only mechanical disruption of the membrane. After recovering the modified cells could be expanded or re-introduced without expansion.
Expansion
Of all the unit operations, cell expansion technologies have undergone the greatest development for cell therapy applications. These bioreactors are typically designed around cell attributes such as suspension or adherence and scale of culture for early or late state process requirements [12].
Unlike the monoclonal antibody biologics industry, cell therapy manufacturing for T cells has not adopted an expansion technology of choice. While 50 liter and larger stirred-tank bioreactors were initially required to produce monoclonal antibodies at a sufficient scale, for autologous application using donor T cells requires fewer cells. Allogeneic off-the-shelf treatments are still in very early development stages. Until dosing requirements are fully understood smaller bioreactors will remain the norm. Currently, the BioBLU® single-use vessel (Eppendorf) and the 3 liter Green CR0003L200 single-use 3 liter stirred-tank bioreactor (Millipore/ Sigma) are small (
The rocking bag used by the GE Wave and Xuri is popular in the 1–5 liter versions. The wave motion of these bioreactors enable efficient gas transfer to allow cell concentrations, and thereby process efficiency, to increase over those found in manual processing. Other wave motion bioreactors found on the market are Finesse SmartRocker (Finesse), the Allegro line of systems (Pall) and Biostat® RM (Sartorius).
The Quantum® Cell Expansion System from TerumoBCT is a hollow fiber bioreactor capable of expanding both adherent and suspension cells with a scale-out potential capable of stocking the bioreactor farms predicted for patient-specific autologous expansions. Proof of concept T cell expansion as well as viral transduction have been demonstrated using the Quantum [36,37]. The hollow-fiber bioreactor enables the most efficient means of gas transfer to the expanding cell population and the semi-permeable membrane allows development of culture feeding strategies to conserve costly media reagents.
Other technologies include the Cocoon platform designed by Octane Biotech. The Cocoon platform has been pioneered at Lonza where proof of concept work has been completed on a T cell expansion including activation, viral transduction, expansion, and wash and concentrate. Unit operations are all performed in the self-contained unit [20]. The CliniMACS Prodigy (Miltenyi Biotec) is also an all in one T cell expansion and purification system that is capable of cell separation, cell expansion, and cell wash and concentrate. Prodigy has a 400-ml volume chamber for cell expansion and is aimed at autologous T cell applications. The G-Rex® from Wilson Wolf has simplified autologous expansion of T cells by placing a gas permeable membrane at the bottom of the vessel. This allows gas transfer to take place where the cell population resides and enables large volumes of media to be added to the vessel; minimizing the need for media exchange. G-Rex may represent a cost effective solution but the level of automation does not compare to the Cocoon, Quantum or Xuri.
With the increased use of pre-filled media bags and sterile welders, media bottles, especially the 0.5 liter variety, will disappear from the manufacturing lab of the future. Not only will this save time and tedium in a biological safety cabinet but, as demand increases and connections are standardized pre-filled media bags will become the norm. Currently, Gibco (ThermoFisher Scientific) and Miltenyi Biotec have marketed their cell culture media in pre-filled bags.
Some believe that for cell therapy manufacturing to become commercially viable, entirely new methods of cell therapy manufacturing need to be developed. Moving manufacturing and testing processes to clean rooms or to controlled non-classified (CNC) spaces in which multiple patient samples can be handled simultaneously will be needed to facilitate high volume production [11].
Big data is a current mantra around cell therapy manufacturing. When the ‘process is the product’, any measurable change in the culture should be recorded and corrected if needed. Process analytical technologies are using the pH and dissolved oxygen levels in stir tank and wave-motion bioreactors to initiate batch feeding schemes. Measurement of metabolites such as glucose and lactate are typically performed manually off-line by devices such as the BioProfile® 100plus (Nova Biomedical) or the YSI 7100 (YSI Life Sciences) and require samples from 50–500 ml. Current probes for real-time measurements are hydrogel based and are easily confounded by media formulations containing proteins. Until more accurate probes exist, closed-loop automation of cell expansion will not be possible as the process will require off-line testing.
In the meantime, cell manufacturing protocols will need to rely on robust culture maintenance device settings provided by process development activities. The biggest change to the cell therapy lab of the future may be the complete elimination of the cell expansion step. At some point, in the not too distant future, the potency of a patients own genetically modified cells may be sufficiently high to eliminate the need for the expansion step altogether, e.g., there has been a recent report of single CAR-T cell being able to induce remission in a chronic lymphocytic leukemia (CLL) patient [38].
Harvest & downstream processing
Automation of the harvesting process results in a greater uniformity of the steps like cell activation and modification as well as maintaining a higher cell viability usually due to shorter handling times.
A number of dedicated cell wash and concentrate platforms exist such as the Sefia Cell Processing System (GE), kSep® technology (Sartorius), the Lovo Automated Cell Processing System (Fresenius Kabi), and the X-Wash (SynGen). Several devices used in upstream processing may be repurposed for cell wash and concentrate like the CliniMACS Plus (Miltenyi), and the Cell Saver-5 (Haemonetics). Finally, TerumoBCT is developing a next-generation cell processing system that will wash and concentrate both autologous and allogeneic batch sizes and will incorporate debeading and elutriation functionality as well. TerumoBCT is also developing an automated closed single-use fill and finish device, Finia, which will allow cryo-protectant mixing and aliquot capability into 4 bags. Other fill–finish technologies include: SmartMax (GE/Biosafe) and for vial-based products, CellSeal (Cook Regentec) and the Crystal® range (Aseptic Technologies).
Regulatory lot release
A discussion of the complete regulatory requirements for the manufacture of CAR-T cells is beyond the scope of this article. A reasonable summary of the process has been published [30].
In the current CAR-T market, neither the cytotoxicity of the treatment as measured by chromium release nor the release of interferon gamma, are predictive of overall clinical success or safety. Both assays fail to examine the extreme diversity of the T cell population [24]. The authors speculate that there may be thousands of unique T cell phenotypes in an expanded PBMC product.
Potency assays of the future should ideally measure clinically relevant functions. In fact, the FDA recommends a panel of assays designed to measure quality, consistency and stability as well as potency [24].
Cold chain
Cell therapy products are living biological materials which, by nature, have a short shelf life. A cold supply chain is critical to delivery of the cells. Cryopreservation of cell therapy materials may be essential for delivery of the product to the hospital for delivery to the patient but it could also be useful in limiting the number of manufacturing sites and overhead costs if centralized or regional manufacturing centers are used for production [11].
Ultra-cold storage is typically required for gene therapy products that need to be stored at -70oC and cell therapy products that need to be stored in the vapor phase of liquid nitrogen below -130oC [21]. It is possible to use a data-logger to chronicle the condition of the cells in liquid nitrogen as they transit from manufacturing to hospital.
Cold and ultra-cold supply chains are expensive. The ability to deliver cell and gene therapy products that can be stored and transported at room temperature or refrigerated (at 2–8oC) could ease the regulatory burden and reduce cost [21].
Even di-methyl sulfoxide (DMSO) could be eliminated from the lab of the future. Some tubing sets (such as those made with polyvinyl chloride [PVC]) are not compatible with sterile welding in the presence of DMSO and prolonged exposure to DMSO may be cytotoxic [28].
Liquid nitrogen cryopreservation is the current gold standard in cell therapy. Currently, commercial-grade liquid nitrogen is not sterile and recently EU guidelines recommend that cell therapy products be stored in the vapor stage to prevent cross-contamination. Interestingly, an alternative method of cryopreservation has been introduced which does not require liquid nitrogen but cools using a Stirling engine or Stirling cryocooler. (EF600, Asymptote/GE). Multi-step cooling profiles are possible using the Stirling cryocooler and results compare to traditional liquid nitrogen systems [39].
Infusion
Once the patients’ dose of cells has been delivered to the hospital or clinical site, the cells will need to thaw prior to being injected. Just as a controlled-rate freezer was used to preserve the cells, a controlled device to thaw the cells and hold them at a pre-programmed temperature until delivered to the patient would eliminate the risks of contamination from water baths and processing variables associated with manual thawing. Process devices capable of handling even more complex final processing of the cells prior to patient delivery will be developed to remove human error and improve product quality and viability. Several controlled thaw devices are available. Asymptote VIA Thaw SC2 (Asymptote/GE) and BioCision ThawSTAR Automated Cell Thawing System (BioCision) are designed to dry thaw vials while the Asymptote VIA Thaw CB1000, Medcision ThawCB and the Sarstedt SAHARA system other are capable of thawing bags [28].
Conclusions
The lab of the future will certainly reflect the current trends in bioprocess manufacturing of autologous CAR-T lymphocytes. Single-use technologies in closed systems will be capable of processing multiple patients’ samples in the same facility.
Eventually, the path from the bench to the clinic will require automation if only to meet cGMP process criteria and manage costs. Automation of the process will likely proceed in a step wise fashion concentrating on the unit operations that are the most labor intensive. Along the way, a deeper understanding of process limits may be revealed by continuous monitoring of the process using process analytical technologies (PAT).
Autologous CAR-T cell manufacture will be handled at a separate manufacturing facility. Transportation of a patient’s cells from the collection site to either a centralized or a regional manufacturing site will place high demands on a documentation and tracking system. Developing a robust cold chain for transporting live cells to the manufacturing facility and returning CAR-modified T cells to the patient will add complexity to an already complex process.
Automation will depend upon the development of new technology solutions. Closed-loop process automation will be possible only when the sampling probe technology for metabolites such as glucose and lactate are robust and reproducible. Current protocols rely upon pH and dissolved oxygen to trigger feeding cycles. Real-time sampling using automated metabolite testing will allow for the refinement of closed-loop process automation steps like cell expansion. Tool companies are already offering automated cell wash and concentrate and fill finish devices. Automated thaw devices will further refine the delivery process. New and automated potency assays will be able to probe the diversity of T cell populations. Ultimately, a fully automated factory complete with electronic documentation and manufacturing execution systems will exist. As new and improved cell therapies are developed the reagent suppliers and tool companies race to meet the demands of the industry to deliver safe, effective, and affordable treatments.
Financial and Competing Interests Disclosure
The authors are employees of TerumoBCT. The authors have no other relevant affiliations or financial involvement with any organization or entity with a financial interest in or financial conflict with the subject matter or materials discussed in the manuscript. This includes employment, consultancies, honoraria, stock ownership or options, expert testimony, grants or patents received or pending, or royalties.
No writing assistance was utilized in the production of this manuscript.REFERENCES
1. Lipsitz YY, Milligan WD, Fitzpatrick I et al. A roadmap for cost-of-goods planning to guide economic production of cell therapy products. Cytotherapy 2017; 19:1383–1391 CrossRef
2. Milligan B, Nis K & Perry R. ISCT COGs survey: results and red flags (Webinar) International Society of Cellular Therapy. November 5, 2013
https://cdn.ymaws.com/isct.site-ym.com/resource/resmgr/CommunityResources/ISCT_COGs_Survey_Results_and.pdf3. James D. How short-term gain can lead to long-term pain: latest advances in CAR-T cell manufacturing. Cell Gene Ther. Insights. 2017; 271–284.
4. Ohno T. Toyota Production System: Beyond Large-Scale Production (1988) Productivity Press, Portland, Oregon.
5. Lowe J, Oliver N. High-performance manufacturing: Evidence from the Automotive Components Industry. Organization Studies 1997; 18(5): 783–798 CrossRef
6. Sahwan MA, Rahman NA, Deros BA. Case studies on the Implementation of Lean manufacturing in the Automotive Malaysian companies. Applied Mechanics and Materials 2014; 465–466: 1180–1184 CrossRef
7. Toussaint JS, Berry LL. The Promise of Lean in Health Care. Mayo Clin. Proc. 2013; 88: 74–82. CrossRef
8. Sonnleitner B, Bioprocess automation and bioprocess design. J. Biotechnol. 1997; 52: 175–179 CrossRef
9. Markowitz-Shulmman A, Siobhan A, Hackmann M, Alper J, Beachy SH. Navigating the Manufacturing Process and Ensuring the Quality of Regenerative Medicine Therapies: Proceedings of a Workshop. The National Academies Press. Washington DC, USA (2017). CrossRef
10. Lopes AG, Sinclair A, Frohlich B. Cost analysis of cell therapy manufacture: autologous cell therapies, part 1. BioProcess Int. 2018; 3–9.
11. Hampson B, Ceccarelli J. Factories of the future: can patient-specific cell therapies get there from here? BioProcess Int. 2016; 4–7.
12. Brindley DA, Wall IB, Bure KE. Automation of cell therapy biomanufacturing minimizing regulatory risks and maximizing return on inverstment. BioProcess Int. 2013; 18–25.
13. Lannon KA, Smith JA, Bure K, and Brindley DA. Quantitative Risk Assessment of Bioaccumulation Attributable to Extractables and Leachables. BioProcess Int. 2015; 13(10) 28–37.
14. Mire-Sluis A, Ma S, Marcovic I Extractables and leachables: challenges and strategies in biopharmaceutical development. BioProcess Int. 2016; Suppl 14: 1–7.
15. Mason C, Hoare M. Regenerative medicine bioprocessing: the need to learn from the experience of other fields. Regen. Med. 2006; 615–623.
16. Ball O, Robinson S, Bure K, Brindley DA, McCall D. Bioprocessing automation in cell therapy manufacturing: outcomes of special interest group automation workshop. Cytotherapy 2018; 592–599.
17. Harris IR, Meacle F, Powers D. Automation in cell therapy manufacturing. BioProcess Int. 2016; 18–21.
18. Tatlock, R. Manufacturing process automation; finding a path forward. BioProcess Int. 2012; 12–15.
19. Levine B. Progress and challenges in the manufacturing of CAR-T cell therapy. Cell Gene Ther. Insights. 2017; 255–260. CrossRef
20. Morrissey JB, Shi Y, Trainor N. End-to-end cell therapy automation: an immunotherapy case study. BioPharm International Development Strategies for Emerging Therapies eBook. 2017; 10–18.
21. Mirasol F. Overcoming hurdles in emerging therapy development. BioPharm International Development Strategies for Emerging Therapies eBook. 2017; 4–9.
22. Brindley DA, French AL, Baptista R et al. Cell therapy bioprocessing technologies and indicators of technological convergence. BioProcess Int. 2014; 14–20.
23. Lopes AG, Sinclair A, Frohlich B. Cost analysis of cell therapy manufacture: autologous cell therapies, part 2. BioProcess Int. 2018; 12–19.
24. K assim SH. Toward an integrated model of product characterization for CAR-T cell therapy drug development efforts. Cell Gene Ther. Insights 2017; 227–237. CrossRef
25. Ramos T, Wang W, Okhovat J, McPherson G, Burger S Human-derived raw materials: Controlled, consistent collections enable successful manufacturing of cell-based regenerative medicine products. J. Immunol. 2014; 192(1 Supplement): 69.15 CrossRef
26. Stroncek DF, Ren J, Lee DW et al. Myeloid cells in peripheral blood mononuclear cell concentrates inhibit the expansion of chimeric antigen receptor T cells. Cytotherapy 2016; 893–901. CrossRef
27. Egelston C. Positive Selection vs Negative Selection for Cell Isolation. PBMC Basics 2013.
28. Iyer RK, Bowles PA, Kim H, Dulgar-Tulloch A. Industrializing autologous adoptive immunotherapies: manufacturing advances and challenges. Front. Med. 2018; 150. CrossRef
29. Imai C, Iwamoto S, Campana D. Genetic modification of primary natural killer cells overcomes inhibitory signals and induces specific killing of leukemic cells. Blood 2005; 106: 376–383. CrossRef
30. Bartido SM. The regulation of CAR-T cells: Latest advances in CAR-T cell manufacturing. Cell Gene Ther. Insights 2017; 239–253. CrossRef
31. Mali P, Yang L, Esvelt K M, Aach J et al. RNA-guided human genome engineering via Cas9. 2013; Science 339(6121), 823–826. CrossRef
32. Carlsten M, Childs RW. Genetic Manipulation of NK Cells for Cancer Immunotherapy: Techniques and Clinical Implications. Front. Immunol. 2015 6, 266. CrossRef
33. Sharei A, Zoldan J, Adamo A et al. A vector-free microfluidic platform for intracellular delivery. Proc. Natl Acad. Sci USA 2013; 110(6), 2082–2087. CrossRef
34. Fratantoni JC, Dzekunov S, Singh V, Liu LN. A non-viral gene delivery system designed for clinical use. Cytotherapy 2003; 5: 208–210. CrossRef
35. Andreoni CC, Altmann T, Bergschneider E et al. Generation of a highly functional cross presenting monocyte-ervired dendritic cells with a new automated and closed electroporation system. J. Immunol. 2018; 200(1 Supplement): 181.4.
36. Nankervis B, Jones M, Vang B et al. Optimizing T Cell Expansion in a Hollow-Fiber Bioreactor Curr. Stem Cell Rep. 2018; 4(10): 45–51. CrossRef
37. Tirughana R, Metz MZ, Li Z, Hall C, GMP Production and Scale-up of Adherent Neural Stem Cells with a Quantum Cell Expansion System. Mol. Ther. Methods Clin. Dev. 2018; 10: 48–56. CrossRef
38. Fraietta JA, Nobles CL, Sammons MA et al. Disruption of TET2 promotes the therapeutic efficacy of CD19-targeted T cells. Nature 2018; 558(7709): 307–312. CrossRef
39. Massie I, Selden C, Morris J et al. Cryopreservation of encapsulated liver spheroids using a cryogen-free cooler: High functional recovery using a multi-step cooling profile. CryoLetters 2011; 32, 158165.
Affiliations
Jim Beltzer Ph.D.
Senior Cell Processing Specialist Cell Processing Terumo BCT jim.beltzer@terumobct.comBrian Nankervis
Scientific Project Lead
Terumo BCTWenyan Leong
Cell Processing Specialist Cell Processing Terumo BCT