Lentiviral vectors in hematopoietic stem cell therapies: mainstay technology, or simply a bridge to gene editing?
Cell Gene Therapy Insights 2018; 4(10), 925-932.
10.18609/cgti.2018.092
Hematopoietic stem cells (HSCs) are quiescent progenitors to a range of blood and immune cell lineages with the propensity to repopulate these cellular niches. This makes them attractive as a cell-based therapy for lysosomal storage disorders [1,2], hemoglobinopathies [3] and primary immune deficiencies [4,5]. Indeed, allogenic identical HLA-matched HSC engraftment has been a mainstay therapeutic approach in the field since the 1960s. However, such cases of HLA matches are rare and finding suitably matched donor’s remains challenging. Furthermore, in many cases of allogenic HSC transplantation, acute graft versus host disease (GVHD) remains a significant concern. This has fuelled the shift towards use of autologous HSC engraftment, in which the patient’s own cells have functional gene expression restored ex vivo before reintroduction.
Hematopoietic stem cells (HSCs) are quiescent progenitors to a range of blood and immune cell lineages with the propensity to repopulate these cellular niches. This makes them attractive as a cell-based therapy for lysosomal storage disorders [1,2], hemoglobinopathies [3] and primary immune deficiencies [4,5]. Indeed, allogenic identical HLA-matched HSC engraftment has been a mainstay therapeutic approach in the field since the 1960s. However, such cases of HLA matches are rare and finding suitably matched donor’s remains challenging. Furthermore, in many cases of allogenic HSC transplantation, acute graft versus host disease (GVHD) remains a significant concern. This has fuelled the shift towards use of autologous HSC engraftment, in which the patient’s own cells have functional gene expression restored ex vivo before reintroduction.
Lentiviral (LV) vectors based on human immunodeficiency virus Type-I (HIV-1) have held long-term value in regenerative medicine, due to their ability to permanently integrate therapeutic genes into dividing and non-dividing cells. LV-mediated HSC therapies have been applied successfully in the clinic for a range of disease indications [6-9], with genomic integration patterns showing to be relatively safe, with minimal insertions near proto-oncogenes and clonal expansions [10,11]. However, although further engineering of LV vector technology [12] and alternative retroviral vectors [13,14] may help maintain their future development in HSC therapies, their scientific novelty is rapidly diminishing. As such, there is a growing trend towards replacing LV vectors with gene editing technologies in preclinical development, with the long-term picture suggesting that LV will be almost wiped-out in years to come (Figure 1). This review will comment on the technological advantages and limitations of gene editing therapies, with respect to how their burgeoning popularity could impact on the future use of LV vectors in autologous HSC therapeutics.
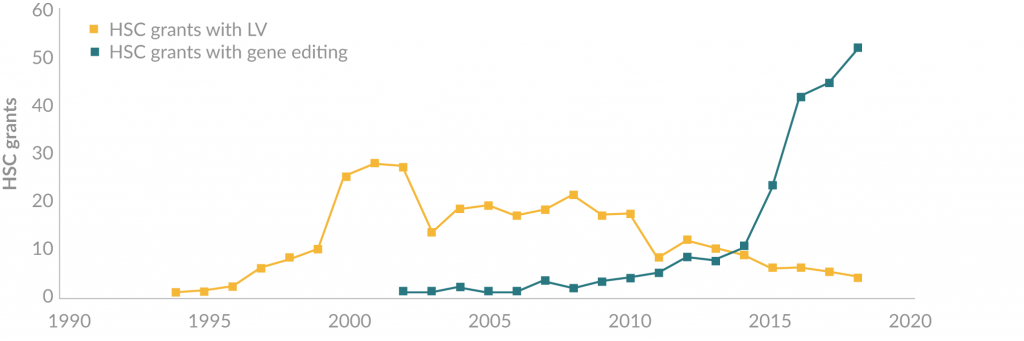
Figure 1. The emergence of gene editing technologies in HSC therapies. Grant statistics obtained from Grantome.com show that since the turn of the decade, LV vectors have steadily been replaced by gene editing technologies in preclinical development of HSC therapies.
Clinical translation of gene editing in HSC therapies: rapid progress, but significant challenges remain
Conventional gene editing utilizes designer endonucleases that can be targeted to and directly cleave the genome at defined sequences, of which there are four main classes: Meganucleases (MGN) [15,16], Zinc Finger Nuclease (ZFN) [17], Transcriptional Activator Like effector Nucleases (TALENs) [18] and the most current Clustered Regularly Interspaced Palindromic Repeat/ Cas9 (CRISPR/Cas9) (for detailed review see [19]). Through the exploitation of diverse cellular DNA repair pathways, the double stranded break (DSB) induced by these reagents can then be resolved in a manner that directly modifies the patient’s genome and by extension the disease-causing mutation. Two canonical cellular DNA damage pathways that can be exploited to enable gene correction, addition and deletion or disruption include: non-homologous end joining (NHEJ) characterized by direct ligation of two DNA termini with intervening small sequence insertions and deletions (InDels), and homology-directed repair (HDR) in which precise modification of the DNA can be achieved through the introduction of a DSB with an exogenous repair template [20].
Gene editing of autologous HSCs has rapidly progressed over the last decade, with ZFN-edited HSCs now in clinical trial for treatment of HIV and sickle cell disease [21,22]. CRISPR/Cas9 has steadily become a dominant tool in preclinical development of HSC therapies, but its potential for broad application in the clinic has recently faced significant questions.
A recent preprint has highlighted a potentially important limitation of CRISPR/Cas9 therapies, in which anti-Cas9 were detected in healthy human sera [23]. Antibodies against Staphylococcus aureus Cas9 and Streptococcus pyogenes Cas9 were detected in 79% and 65% of samples, respectively. Additionally, anti-Cas9 T cell responses were detected at rates of 46% to saCas9. This undoubtedly has important implications for systemic expression of Cas9 for body-wide, in vivo gene editing, although its relevance to ex vivo HSC therapies is of less concern if Cas9 expression can be restricted to transient duration prior to transplantation. Thus, the delivery system of gene-editing reagents and the resultant expression profile remain continual and ongoing considerations for developing therapeutic strategies. As such, Cas9 has been delivered to HSCs as plasmid (DNA) [24], mRNA [25] and Ribonucleotide protein complexes [26]. The shift towards the use of ribonucleotide proteins (RNPs) in the field is occurring in the pursuit of ‘hit and run’ gene editing, in which the expression of the Cas9 protein is high albeit transient.
Additionally, recent data has highlighted that deleterious genotoxic events could occur in some gene editing scenarios, where large deletions and chromosomal rearrangements followed DSB repair, leading to chromosomal instability [27]. Damage associated with DSBs could be minimized through stringent design and validation of single-guide RNAs (sgRNAs), to minimize ‘off-target’ effects, which can occur at unintended genomic sites containing up to six mismatches to the sgRNA sequence [28]. Multiple strategies are being employed to address this, such as empirical research to aid and inform in silico guide design [29-31] and a multitude of genome wide off-target assessment methods such as guide-seq [28], digenome-seq [32] and circle-seq [33] to permit judicious sgRNA selection. Protein engineering of the Cas9 endonuclease has also been undertaken and has resulted in numerous variants, including nickases [34], Cas9-fokI fusions [35] and high-fidelity variants with ‘neutralizing base’ substitutions at the RNA-DNA interface [36,37].
Optimizing the delivery and specificity of current gene editing technologies are of crucial importance to efficient therapeutic gene editing, but a further technical challenge lies in the scalability of this approach and biasing the cellular DNA repair pathway to the appropriate DSB resolution pathway. Many therapeutic HSC strategies are reliant upon HDR to achieve genetic correction, which is a particularly inefficient process in quiescent HSCs, due to HDR and NHEJ working antagonistically throughout the cell cycle. A multitude of approaches have sought to bias cellular DNA correction to a HDR repair profile, including small molecule inhibitors that target and supress range of protein mediators throughout the NHEJ DNA repair pathway (reviewed in [38]), cellular synchronisation to late G2/S phases [39] and overexpression of HDR protein mediators [40,41]. However, the long-term effects of these pharmacological treatments on the propensity of HSC to undergo self-renewal and differentiation remains to be determined. In addition, integration methods independent of conventional HDR are subject of on-going investigation and studies including homology independent transgene integration (HITI) [42], microhomology end joining (MMEJ) [43] and Rad51 independent single strand annealing (SSA) integration [44].
An alternative method being explored for HSC gene editing is ‘base editing’, which theoretically would not be restricted to particular phases of the cell cycle. Base editing is mediated through delivery of Cas9 orthologues fused to enzymes that catalyze single nucleotide mutations. The first generation of these utilized APOBEC-mediated cytidine deamination to affect a C•G to T•A transition [45] and was later expanded to adenine deaminases with the ability to convert A•T to G•C base pairs [46]. The main advantage of the technology is that resulting genomic lesions are primarily composed of single-stranded ‘nicks’ and thus less deleterious to the genome than full DSBs. Notably, low level DSB events do occur with this system, inspiring further optimisation of base editors by limiting DSB formation with additional modifications [47] and directed evolution strategies [46]. Furthermore, fusion of these base editing enzymes to other Cas9 orthologues has served to further expand the scope of potential sites that could be edited and improve amenable delivery strategies [48].
As outlined previously, LV technology has been successfully applied in scenarios suited to unregulated expression of the therapeutic transgene. However, as HSC gene therapy expands though an increasing range of disease indications, it may be necessary to incorporate more sophisticated transcriptional control over transgenes that may not be appropriate to constitutive expression. Although this has been successfully applied to treatment of beta-thalassemia, the use of complex non-coding sequences in some vectors has led to aberrant transcriptional effects in clinical trials [49]. Harnessing of endogenous gene regulation is likely to present a favorable approach for the treatment of the majority of disease indications. But in some cases, supraphysiological expression of the transgene product is an important feature of LV-HSC therapies, in order to provide adequate cross-correction of the required tissues [50-53]. In these cases, endogenous gene expression levels may not be sufficient, meaning that conventional gene editing would be unfavorable.
Translational gene editing also faces complex logistical challenges, given that an entire preclinical development pathway will theoretically be required for each individual genomic target. Additionally, as we have described in previous sections, gene editing therapies have very precise requirements in their design, which currently restricts the application of a single technology to the treatment of all patient genotypes for a given disease indication. Indeed, CRISPR/Cas9 methodologies are far more diverse than LV designs, which could permit treatment of a broad range of genotypes. But this creates a potential headache from a commercial perspective, as a single company would likely need to acquire access to multiple patents in order to treat the majority of patients within a single disease area. Either that, or firms would need to be built around a single CRISPR/Cas9 platform technology and spread its application across a broader range of diseases. Either way, it is likely that commercial gene editing therapies will follow a different path to those incorporating LV.
Concluding remarks
With LV vectors providing the ability to treat the vast majority of patient genotypes, along with growing evidence for clinical safety and efficacy, it is likely that their use will hold long-term value in ex vivo regenerative medicine. But specific diseases and mutations might necessitate use of gene editing in scenarios requiring strict regulatory control over the therapeutic gene. The most likely scenario is that both LV vectors and gene editing technologies will acquire niche applications in the clinic, with their use dictated by the characteristics of a given disease and the common genotypes across patient populations. The shift in popularity towards gene editing in preclinical studies can of course be partly explained by the diminishing novelty of LV technology, although the documented clinical success of LV therapies surely means that they will remain an important tool in genomic medicine for years to come.
financial & competing interests disclosure
The authors have no relevant financial involvement with an organization or entity with a financial interest in or financial conflict with the subject matter or materials discussed in the manuscript. This includes employment, consultancies, honoraria, stock options or ownership, expert testimony, grants or patents received or pending, or royalties. No writing assistance was utilized in the production of this manuscript.
References
1. Gomez-Ospina N, Scharenberg SG, Mostrel N et al. Human genome-edited hematopoietic stem cells phenotypically correct Mucopolysaccharidosis type I. bioRxiv 2018; 408757. CrossRef
2. Eichler F, Duncan C, Musolino PL et al. Hematopoietic Stem-Cell Gene Therapy for Cerebral Adrenoleukodystrophy. N. Engl. J. Med. 2017; 377: 1630–8. CrossRef
3. Negre O, Eggimann A-V, Beuzard Y et al. Gene Therapy of the b-Hemoglobinopathies by Lentiviral Transfer of the b(A(T87Q))-Globin Gene. Hum. Gene Ther. 2016; 27: 148–65. CrossRef
4. De Ravin SS, Wu X, Moir S et al. Lentiviral hematopoietic stem cell gene therapy for X-linked severe combined immunodeficiency. Sci. Transl. Med. 2016; 8: 335ra57-335ra57.
5. Pavel-Dinu M, Wiebking V, Dejene BT et al. Gene Correction for SCID-X1 in Long-Term Hematopoietic Stem Cells. bioRxiv 2018: 397463. CrossRef
6. Cartier N, Hacein-Bey-Abina S, Bartholomae CC et al. Hematopoietic Stem Cell Gene Therapy with a Lentiviral Vector in X-Linked Adrenoleukodystrophy. Science 2009; 326: 818–23. CrossRef
7. Biffi A, Montini E, Lorioli L et al. Lentiviral Hematopoietic Stem Cell Gene Therapy Benefits Metachromatic Leukodystrophy. Science 2013; 341: 1233158. CrossRef
8. Sessa M, Lorioli L, Fumagalli F et al. Lentiviral haemopoietic stem-cell gene therapy in early-onset metachromatic leukodystrophy: an ad-hoc analysis of a non-randomised, open-label, phase 1/2 trial. Lancet 2016; 388: 476–87. CrossRef
9. Ribeil J-A, Hacein-Bey-Abina S, Payen E al. Gene Therapy in a Patient with Sickle Cell Disease. N. Engl. J. Med. 2017; 376: 848–55. CrossRef
10. Biffi A, Bartolomae CC, Cesana D et al. Lentiviral vector common integration sites in preclinical models and a clinical trial reflect a benign integration bias and not oncogenic selection. Blood 2011; 117: 5332–9. CrossRef
11. Biasco L, Pellin D, Scala S et al. In Vivo Tracking of Human Hematopoiesis Reveals Patterns of Clonal Dynamics during Early and Steady-State Reconstitution Phases. Cell Stem Cell 2016; 19: 107–19. CrossRef
12. Vink CA, Counsell JR, Perocheau DP et al. Eliminating HIV-1 Packaging Sequences from Lentiviral Vector Proviruses Enhances Safety and Expedites Gene Transfer for Gene Therapy. Mol. Ther. 2017; 9: 10–20. CrossRef
13. Suerth JD, Maetzig T, Brugman MH et al. Alpharetroviral Self-inactivating Vectors: Long-term Transgene Expression in Murine Hematopoietic Cells and Low Genotoxicity. Mol. Ther. 2012; 20: 1022–32. CrossRef
14. Sweeney NP, Regan C, Liu J et al. Rapid and Efficient Stable Gene Transfer to Mesenchymal Stromal Cells Using a Modified Foamy Virus Vector. Mol. Ther. 2016; 24: 1227–36. CrossRef
15. Epinat J-C, Arnould S, Chames P et al. A novel engineered meganuclease induces homologous recombination in yeast and mammalian cells. Nucleic Acids Res. 2003; 31: 2952–62. CrossRef
16. Thierry A, Dujon B. Nested chromosomal fragmentation in yeast using the meganuclease I-Sce I: a new method for physical mapping of eukaryotic genomes. Nucleic Acids Res. 1992; 20: 5625–31. CrossRef
17. Kim YG, Cha J, Chandrasegaran S. Hybrid restriction enzymes: zinc finger fusions to Fok I cleavage domain. Proc. Natl Acad. Sci. USA 1996; 93: 1156–60. CrossRef
18. Miller JC, Tan S, Qiao G et al. A TALE nuclease architecture for efficient genome editing. Nat. Biotechnol. 2011; 29: 143–8. CrossRef
19. Kim H, Kim J-S. A guide to genome engineering with programmable nucleases. Nat. Rev. Genet. 2014; 15: 321–34. CrossRef
20. Ciccia A, Elledge SJ. The DNA damage response: making it safe to play with knives. Mol. Cell 2010; 40: 179–204. CrossRef
21. Chang K-H, Smith SE, Sullivan T et al. Long-Term Engraftment and Fetal Globin Induction upon BCL11A Gene Editing in Bone-Marrow-Derived CD34 + Hematopoietic Stem and Progenitor Cells. Mol .Ther. Methods Clin. Dev. 2017; 4: 137–48. CrossRef
22. DiGiusto DL, Cannon PM, Holmes MC et al. Preclinical development and qualification of ZFN-mediated CCR5 disruption in human hematopoietic stem/progenitor cells. Mol. Ther. Methods Clin. Dev. 2016; 3: 16067. CrossRef
23. Charlesworth CT, Deshpande PS, Dever DP et al. Identification of Pre-Existing Adaptive Immunity to Cas9 Proteins in Humans. bioRxiv 2018: 243345. CrossRef
24. Antoniani C, Meneghini V, Lattanzi A et al. Induction of fetal hemoglobin synthesis by CRISPR/Cas9-mediated editing of the human b-globin locus. Blood 2018; 131: 1960–73. CrossRef
25. Hoban MD, Lumaquin D, Kuo CY et al. CRISPR/Cas9-Mediated Correction of the Sickle Mutation in Human CD34+ cells. Mol. Ther. 2016; 24: 1561–9. CrossRef
26. Wen J, Tao W, Hao S, Zu Y. Cellular function reinstitution of offspring red blood cells cloned from the sickle cell disease patient blood post CRISPR genome editing. J. Hematol. Oncol. 2017; 10: 119. CrossRef
27. Kosicki M, Tomberg K, Bradley A. Repair of double-strand breaks induced by CRISPR–Cas9 leads to large deletions and complex rearrangements. Nat. Biotechnol. 2018; 36: 765. CrossRef
28. Tsai SQ, Zheng Z, Nguyen NT et al. GUIDE-seq enables genome-wide profiling of off-target cleavage by CRISPR-Cas nucleases. Nat. Biotechnol. 2014; 33: 187–97. CrossRef
29. Doench JG, Hartenian E, Graham DB et al. Rational design of highly active sgRNAs for CRISPR-Cas9–mediated gene inactivation. Nat. Biotechnol. 2014; 32: 1262–7. CrossRef
30. Doench JG, Fusi N, Sullender M et al. Optimized sgRNA design to maximize activity and minimize off-target effects of CRISPR-Cas9. Nat. Biotechnol. 2016; 34: 184–91. CrossRef
31. Moreno-Mateos MA, Vejnar CE, Beaudoin J-D et al. CRISPRscan: designing highly efficient sgRNAs for CRISPR-Cas9 targeting in vivo. Nat. Methods 2015; 12: 982–8. CrossRef
32. Kim D, Bae S, Park J et al. Digenome-seq: genome-wide profiling of CRISPR-Cas9 off-target effects in human cells. Nat. Methods 2015; 12: 237–43. CrossRef
33. Tsai SQ, Nguyen NT, Malagon-Lopez J, Topkar V V, Aryee MJ, Joung JK. CIRCLE-seq: a highly sensitive in vitro screen for genome-wide CRISPR–Cas9 nuclease off-targets. Nat. Methods 2017; 14: 607–14. CrossRef
34. Ran FA, Hsu PD, Lin C-Y et al. Double nicking by RNA-guided CRISPR Cas9 for enhanced genome editing specificity. Cell 2013; 154: 1380–9. CrossRef
35. Tsai SQ, Wyvekens N, Khayter C et al. Dimeric CRISPR RNA-guided FokI nucleases for highly specific genome editing. Nat. Biotechnol. 2014; 32: 569–76. CrossRef
36. Kleinstiver BP, Pattanayak V, Prew MS et al. High-fidelity CRISPR–Cas9 nucleases with no detectable genome-wide off-target effects. Nature 2016; 529: 490–5. CrossRef
37. Slaymaker IM, Gao L, Zetsche B, Scott DA, Yan WX, Zhang F. Rationally engineered Cas9 nucleases with improved specificity. Science 2016; 351. CrossRef
38. Velic D, Couturier AM, Ferreira MT et al. DNA damage signalling and repair inhibitors: The long-sought-after achilles heel of cancer. Biomolecules 2015; 5: 3204–59. CrossRef
39. Lin S, Staahl B, Alla RK, Doudna JA. Enhanced homology-directed human genome engineering by controlled timing of CRISPR/Cas9 delivery. Elife 2014; 3: e04766. CrossRef
40. Di Primio C, Galli A, Cervelli T, Zoppè M, Rainaldi G. Potentiation of gene targeting in human cells by expression of Saccharomyces cerevisiae Rad52. Nucleic Acids Res. 2005; 33: 4639–48. CrossRef
41. Wang L, Yang L, Guo Y et al. Enhancing Targeted Genomic DNA Editing in Chicken Cells Using the CRISPR/Cas9 System. PLoS One 2017; 12: e0169768. CrossRef
42. Suzuki K, Tsunekawa Y, Hernandez-Benitez R et al. In vivo genome editing via CRISPR/Cas9 mediated homology-independent targeted integration. Nature 2016; 540: 144–9. CrossRef
43. Sakuma T, Nakade S, Sakane Y, Suzuki K-IT, Yamamoto T. MMEJ-assisted gene knock-in using TALENs and CRISPR-Cas9 with the PITCh systems. Nat. Protoc. 2015; 11: 118–33. CrossRef
44. Richardson CD, Ray GJ, DeWitt MA, Curie GL, Corn JE. Enhancing homology-directed genome editing by catalytically active and inactive CRISPR-Cas9 using asymmetric donor DNA. Nat. Biotechnol. 2016; 34. CrossRef
45. Komor AC, Kim YB, Packer MS, Zuris JA, Liu DR. Programmable editing of a target base in genomic DNA without double-stranded DNA cleavage. Nature 2016; 533: 420–4. CrossRef
46. Gaudelli NM, Komor AC, Rees HA et al. Programmable base editing of A•T to G•C in genomic DNA without DNA cleavage. Nature 2017; 551: 464–71. CrossRef
47. Komor AC, Zhao KT, Packer MS et al. Improved base excision repair inhibition and bacteriophage Mu Gam protein yields C:G-to-T:A base editors with higher efficiency and product purity. Sci. Adv. 2017; 3: eaao4774.
48. Kim YB, Komor AC, Levy JM, Packer MS, Zhao KT, Liu DR. Increasing the genome-targeting scope and precision of base editing with engineered Cas9-cytidine deaminase fusions. Nat. Biotechnol. 2017; 35: 371–6. CrossRef
49. Cavazzana-Calvo M, Payen E, Negre O et al. Transfusion independence and HMGA2 activation after gene therapy of human b-thalassaemia. Nature 2010; 467: 318–22. CrossRef
50. Ashley GA, Desnick RJ, Gordon RE, Gordon JW. High Overexpression of the Human a-Galactosidase A Gene Driven by Its Promoter in Transgenic Mice: Implications for the Treatment of Fabry Disease. J. Investig. Med. 2002; 50: 185–92. CrossRef
51. Biffi A, Capotondo A, Fasano S et al. Gene therapy of metachromatic leukodystrophy reverses neurological damage and deficits in mice. J. Clin. Invest. 2006; 116: 3070–82. CrossRef
52. Biffi A, Montini E, Lorioli L et al. Lentiviral Hematopoietic Stem Cell Gene Therapy Benefits Metachromatic Leukodystrophy. Science 2013; 341: 1233158. CrossRef
53. Langford-Smith A, Wilkinson FL, Langford-Smith KJ et al. Hematopoietic Stem Cell and Gene Therapy Corrects Primary Neuropathology and Behavior in Mucopolysaccharidosis IIIA Mice. Mol. Ther. 2012; 20: 1610–21. CrossRef
Affiliations
Marc Moore
Dubowitz Neuromuscular Centre, Molecular Neurosciences Section, Developmental Neurosciences Programme, UCL Great Ormond Street Institute of Child Health,
30 Guilford Street,
London,
UK
and,
NIHR Great Ormond Street Hospital Biomedical Research Centre,
30 Guilford Street,
London
WC1N 1EH, UK
John R Counsell
Dubowitz Neuromuscular Centre,
Molecular Neurosciences Section,
Developmental Neurosciences Programme,
UCL Great Ormond Street Institute of Child Health,
30 Guilford Street, London,
UK
and,
NIHR Great Ormond Street Hospital Biomedical Research Centre,
30 Guilford Street,
London WC1N 1EH, UK
This work is licensed under a Creative Commons Attribution- NonCommercial – NoDerivatives 4.0 International License.