Automated Cell Expansion: Trends & Outlook of Critical Technologies
Cell Gene Therapy Insights 2018; 4(9), 851-871.
10.18609/cgti.2018.086
Cell therapy products (CTP) are set to experience a surge in demand by the mass market in the coming decade. Automation is expected to play a key role in meeting this demand. In this review, we summarize the features of current automated cell expansion systems and highlight some of the trends and promising developments. The focuses of current systems are enabling scalability, maintaining sterility, eliminating contamination and controlling quality. To attain full automation of CTP manufacturing, several challenges have to be overcome. We identify challenges pertaining to scalability via sterile reconnections, direct cell measurements for cell-specific quality control, logistical issues in traceability, temperature control during transport, as well as administrative burden of regulations. Ultimately, automation would ensure product quality and repeatability at a lower labor cost.
Submitted for Peer Review: Aug 24 2018 Published: Nov 20 2018
Cell Therapy Product Manufacturing
The US Pharmacopeial Convention defines cell therapy as a treatment in which whole cells are administered to a patient, often after a modification and expansion process in a lab, to treat a disease, condition or injury [1]. A subset of cell therapies involves the modification of the cells’ genetic material before administration and they are specifically termed gene-modified cell therapies [2]. In this review, for clarity, we refer to both pure cell therapies and gene-modified cell therapies collectively as cell therapies. In cell therapy, cells administered may either remove diseased cells [3], or directly regenerate or replace damaged tissues [4,5], or exert paracrine effect to achieve therapeutic outcomes [6,7]. This offers a potentially more effective treatment for diseases that currently have poor prognosis [8] and demand for cell therapy is expected to surge in the next decade as more products enter early and late-phase clinical trials [9]. In particular, immuno-oncology is leading in the number of clinical trials, with the chimeric antigen receptor (CAR) T-cell therapy alone accounting for over 100 clinical trials in progress in 2016, which is a 250% increase from the previous year, and with almost $600 million in venture capital equity [10].
For the cell therapy community, year 2017 was an exciting year as two autologous CAR T-cell therapy products were approved by the US FDA. The first CAR T-cell therapy to be approved is Kymriah by Novartis (Novartis International AG, BS, Switzerland). Kymriah is an autologous CD19-directed CAR T-cell immunotherapy treatment and received two FDA approvals. Kymriah is indicated for all patients with B cell precursor acute lymphoblastic leukaemia (ALL) that is refractory or in second or later relapse [11]. The second CAR T-cell therapy approved is the Yescarta by Kite Pharma (Gilead Sciences Inc., Foster City, CA, USA). Yescarta is an autologous CD19-direct CAR T-cell immunotherapy for adult patients with large B-cell lymphoma [12].
Cell therapy is further categorised as allogeneic and autologous cell therapies. They undergo the same steps in manufacturing, and differ only in the source of the cells. Allogeneic cell therapy product (CTP) is CTP that is derived from healthy donor cells and is designed to be universally suitable for all patients. Donor cells are selected for superior qualities such as the ability to persist in the patient, the potency of CTP derived from it, and the ability to survive the cryopreservation process. Allogeneic CTP is thus expected to be available ‘off-the-shelf’ and enable critically ill patients to receive treatment within a shorter time period. In contrast, autologous cell therapy requires more time as it starts from the patient’s own cell sample, modifying and expanding to the target number of cells for a therapeutic dose. While there is significantly lower risk of developing graft-versus-host disease (GvHD) [13], patients may not have sufficient healthy or viable cells to produce the CTP. Nevertheless, autologous CTP is an attractive option as it reduces complications from immune rejection and certain CTPs appear to have higher efficiency when autologous T cells were used [14].
Manufacturing process & requirements
While the details of each step in the manufacturing process for different CTP differ, they can generally be categorized under isolation, modification, expansion, harvest and cryopreservation as illustrated in Figure 1 . Modification includes the maturation and specialisation of cells via exposure to biological molecules such as cytokines, growth factors and feeder cells, and direct genetic modification in gene-modified cell therapy. Protocols often differ in the sequence and duplication of the steps, with the isolation, modification and expansions steps are the most variable steps. Some processes may have repeated cycles of isolation and expansion to increase the presence of the target cell population while others have an additional isolation step before harvesting. Minimally manipulated cell therapy, usually involving stem cells, consists of only three steps, namely harvest, purification and infusion, which can be done within a day at the bedside [15,16]. These therapies therefore do not requirement extensive manufacturing support. We focus, instead, on cell therapies with manufacturing processes that involve modification and/or expansion of the seed cell population. For instance, in the manufacturing of autologous CAR T-cells, T cells are isolated from patient’s whole blood and modified to express the CAR recombinant protein. This transmembrane protein has both the antigen-specificity of an antibody and the ability to activate T cells directly, allowing the CAR T-cells to not only locate but also destroy the target cells. The transfected CAR T-cells are then expanded to the required cell count or therapeutic dose, harvested and finally cryopreserved until the patient is ready for the treatment. A review of different manufacturing protocols for CAR T-cells is available here [17].
The current good manufacturing practice (cGMP) manufacturing process for CTP is fraught with logistical and manpower challenges. In addition to the difficulty of sourcing cGMP raw materials and ancillary materials, the large number of skilled specialists needed and the requirement for aseptic environment incur a high cost for manufacturers. The most labor-intensive and time-consuming step is arguably the expansion step. This step is variable according to protocols, but usually spans several weeks [18–20] as the initial number of seed cells is small and cells take time to grow to therapeutic dose even under optimal conditions. Specialists spend significant time to perform multiple expansions of the cell culture into increasingly bigger cell culture vessels. Towards the end of the expansion step, each specialist may be handling hundreds of standard cell culture vessel every day. It is not surprising then that an economic model estimated the cost of cell therapy for one patient to be at least US$100,000 [21] with labor cost as the largest expenditure. In addition, a significant portion of the current manufacturing process involves open manipulation of the cell culture, especially during expansion, and has to be performed in areas with cleanroom Class 100 designation to comply with cGMP guidelines [22]. As labor-intensive expansion step can only support small-scale development phases, they will fall behind in meeting future demand for CTPs.
Automated manufacturing
Thus, the cell therapy community is increasingly calling for the development of automated and/or closed systems [23]. Automated systems are expected to reduce the overall cost and manpower demand in CTP manufacturing by reducing the need for human intervention as well as enabling robust and reliable processes [10]. Closed systems protect the cell culture environment from external contamination, which enables manufacturing to take place at lower grade cleanrooms. In addition to potential cost savings, automated and/or closed systems for CTP manufacturing is in line with regulatory guidelines such as the Quality by Design (QbD) approach [24] and the Process Analytical Technology (PAT) framework [25]. QbD and PAT guide CTP developers in establishing processes to repeatably and reliably achieve high quality end products. Issues with meeting the stringent product specifications is a challenge even for established pharmaceuticals giants such as Novartis [26]. Automated systems designed with PAT and QbD in mind will help to reduce product variability by enabling closed and timely monitoring of the cultures.
As of 21 Aug 2018, a search on ClinicalTrials.gov yielded 785 studies involving cell therapy, of which 142 Phase 2, 3 and 4 studies are listed as completed studies. Among the completed Phase 3 studies, CTP doses ranged from 3 x 107 to 1.2 x 109 cells [15,16,18,27,28]. While not all studies will come to fruition, the large number of studies underway points to an imminent need for manufacturers to increase their production capacity. As CTP production increases, the need to reduce cost and manpower requirement has spurred the development of systems that automate one or more steps of the manufacturing process. Nonetheless, the process of automating cell manufacturing is not trivial as many of these steps rely on the dexterity and the experience of the operator. Translating these skills into quantifiable parameters for engineered systems requires a deep understanding of the biological processes and an appreciation for the inherent variations in biological systems and the input cell quality. The simultaneous requirement for sterility and access to the cell culture for monitoring and addition of ancillary materials also poses design challenges. Furthermore, as the manufacturing process is highly varied, automated systems have to accommodate flexibility in the process sequence and order.
In this review paper, we compile commercially available automated cell expansion systems and discuss some of the common features found in these systems. Following this survey of currently available systems, we highlighted key challenges that have to be solved and technical gaps that have to be bridged before CTP manufacturing can be fully automated.
Current Trends in Automated Systems
Cell manufacturing, like all other forms of manufacturing, aims to repeatably produce quality products at a low cost. Considering the high cost of training and retaining highly skilled personnel in CTP manufacturing, automating the manufacturing process becomes the obvious solution. Eventually, manufacturing systems are expected to be fully automated from needle-to-needle, spanning extraction to infusion. Full automation would eliminate costly manpower requirements in the conduct of steps or even the transfer between steps. At this point in time, systems in the market automate either specific steps or a series of steps of the entire manufacturing process, outlined in Figure 1 .
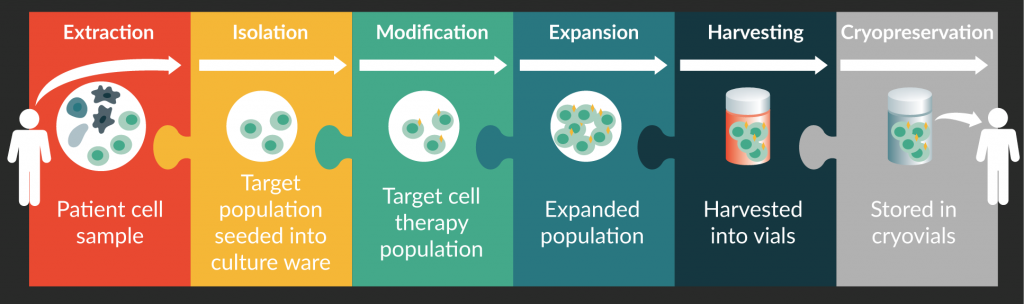
Figure 1. Main steps in cell therapy product manufacturing: extraction, isolation, modification, expansion, harvesting, and cryopreservation.
There are several automated systems currently in the market for CTP manufacturing, targeted for different types of CTP manufacturing. Table 1 summarizes the key technology and features incorporated into each system, and their volumetric capacities. Only systems that are automated, or are in the pipeline for automation, and are indicated for cell therapy manufacturing are considered for the purpose of this review. While the systems are highly varied and designed for different target CTPs, the following subsections highlight some of the prevailing concepts and elements.
Table 1. Summary of technologies in automated systems currently in the market for CTP manufacturing. | ||||||||||||
---|---|---|---|---|---|---|---|---|---|---|---|---|
Company | Product name | Capacity | Scale-up or scale-Out | Development- or production-centric | Key technology | Monitoring capabilities | Cell type(s) supported | Automated steps in CTP manufacturing | ||||
Isolate | Modify and maintain | Expand | Harvest | Cryopreserve | ||||||||
TerumoBCT | Quantum® Cell Expansion System | 21000cm2 | Scale-out | Development and production | 3D, functional closed bioreactor containing ~11,500 hollow fibers | Temperature, pH and DO sensors; sampling port | Adherent and suspension | ✓ | ✓ | ✓ | ✓ | x |
GE Healthcare | Xuri™ Cell Expansion System | 0.3–25 L | Scale-up and scale-out | Development (W5 system) and production (W25 system) | WAVE rocking technology provides mixing and aeration | Temperature, pH, DO, weight sensors | Suspension | x | ✓ | ✓ | ✓ | x |
Pall Life Sciences | Xpansion® Multiplate Bioreactor System | 122400cm2; 1.6–21.9 L | Scale-out | Development and production | 2D multiplate design | Temperature, pH and DO sensors; sampling port | Adherent | x | x | ✓ | x | x |
Pall Life Sciences | XRS 20 Bioreactor System | 2–20 L | Scale-out | Production | Bi-axial agitation that produces a low turbulence, swirling action | Temperature, pH, DO, pressure sensors; sampling port | Suspension | x | x | ✓ | x | x |
Tap Biosystems | ambr® 250 high throughput | 0.1– 0.25L | Scale-out | Development | Automation of design of experiment approaches to process automation | Temperature, pH and DO sensors; sampling port | Suspension | x | x | ✓ | x | x |
ESCO Aster | Tide Cell® using Tide Motion Technology | 2–20 L; 50–100 L; linearly scalable up to 5000 L | Scale-up and scale-out | Development and production | Extended large scale system of the lab scale CelCradle® bioreactor. Compatible with BioNoc™ II carriers | Temperature, pH, DO, aeration, foaming, agitation and level sensors; sampling ports | Adherent | x | x | ✓ | ✓ | x |
ESCO Global | VacciXcell™ Hybrid bioreactor | ≤5 L for adherent culture; ≤6.5 L for suspension culture | Scale-out | Development and production | Capable of supporting both suspension and adherent cells; capacity of up to 11g of BioNoc™ II carriers | Temperature, pH, DO, aeration, foaming, agitation and level sensors; sampling ports | Adherent and suspension | x | x | ✓ | ✓ | x |
Wilson Wolf | G-REX® | 10–500 cm2; 0.04–5.5 L | Scale-out | Production | Gaseous exchange via membrane | Nil | Adherent and suspension | x | x | ✓ | ✓ | x |
Charter Medical | EXP-PAK™ Cell Expansion Bio-Containers | 0.03–1.25 L | Scale-out | Production | Made from a single-web polyolefin gas permeable film; reusable sampling valve | Nil | Suspension | x | ✓ | ✓ | ✓ | x |
Miltenyi Biotec | CliniMACS Prodigy® | 0.05–0.66 L | Scale-out | Production | Magnetic separation; T-cell transduction | Integrated microscope; sampling pouches | Adherent | ✓ | ✓ | ✓ | ✓ | x |
Octane | Cocoon™ | Information not available | Scale-out | Production | Extensive automation and integration | Embedded sensors; automatic delivery of cell samples for external analysis | Adherent and suspension | ✓ | ✓ | ✓ | ✓ | x |
VivaBiocell | NANT-001 | 636 cm2 | Scale-out | Production | Follows manual cell culture principles | Image-based confluency estimates | Adherent and semi-adherent | x | x | ✓ | ✓ | x |
Sartorius Stedim Biotech | CompacT SelecT™ | Up to 90x T175 format flasks and 210 plates | Scale-out | Development and production | An isolator with robot arms | Temperature, pH, DO sensors; cell count, cell viability measurement | Adherent and suspension | x | ✓ | ✓ | ✓ | x |
Merck | Mobius® CellReady 3L bioreactor | 3 L | Scale-out | Development | Single-use stir tank | 3x probe ports for 12 mm PG 13.5 threaded probes | Adherent and suspension | x | x | ✓ | ✓ | x |
*DO: Dissolved oxygen. |
Scale-up & scale-out
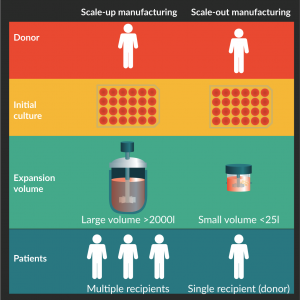
Figure 2. Scale-up and scale-out manufacturing models. Both models undergo the same cell manufacturing steps but adopt different expansion scales.
To meet the growing demands for CTPs, scalability is a necessary progression for manufacturers. As illustrated in Figure 2, two models are appropriate for CTP manufacturing: scale-up model and scale-out model. Scale-up model refers to the increase in volume of the culture vessel, and is typically adopted by biotechnology companies. In this model, cells are selected for traits that enable them to thrive in large culture environment and withstand large volume processes, while still producing quality end products. As many of the CTPs are still in the nascent phase, current automated systems are mostly small-scale systems such as the VacciXcell™ Hybrid Bioreactor (Esco Micro Pte. Ltd, Singapore) and the Quantum® Cell Expansion System (Terumo BCT, Inc., Lakewood, CO, USA). In general, when cell cultures are scaled up, the master cell stock is first adapted into a suspension culture, which is easier to process at large volumes. Unlike in the biopharmaceutical industry, CTPs cannot be modified to a suspension culture as most CTP are based on the innate characteristics of the cells and changes to the cell behavior will require extensive safety verification before it can be infused into patients. Even with solutions such as microcarriers and hollow fibres to increase the cell culture surface area for adherent cells, anecdotally, many adherent cells exhibit different characteristics depending on how they are cultured. This poses a problem when the process is transferred from small scale to large-scale production.
Since CTP dose is relatively small for each patient [15,16,18,27,28], the scale-up model is associated exclusively with allogeneic CTP manufacturing where a universal CTP is developed as an off-the-shelf product suitable for many patients. By contrast, the scale-out model increases the number of small-scale equipment, each of which produces enough cells for a single patient. Autologous CTP are limited to the scale-out model as each batch is intended for the specific patient and autologous CTPs for different patients do not share equipment. Even in cGMP facilities, the cultures are kept in separate rooms to physically prevent cross contamination [29].
The scale-out model is well suited for the manufacturing process of autologous CTP and may also de-risk the manufacture of allogeneic CTP by physically limiting the spread of contamination. For the scale-out model to be cost-effective, the system has to be modular; the cell expansion step may take up to several weeks of culturing [18–20], depending on the quality of the patient’s sample. When all-in-one systems such as the CliniMACS Prodigy® are used in protocols that have long expansion periods, the other functions such as isolation of target cells and harvesting of CTP, cannot be utilized by or shared with other CTPs in the pipeline. This results in redundancy and under-utilized resources. Thus, modular systems would be much more cost-effective for the high-mix-low-volume manufacturing industry.
Often discussed alongside the scale-out model is the concept of de-centralised manufacturing, where manufacturing of CTP is done closer to the bedside. The business model for CTP manufacturing can range from a completely centralized manufacturing where a large geographical area is served by one specialised manufacturing system, to complete de-centralization where manufacturing is done at the hospital starting with either autologous or allogeneic seed cells. Between these two extremes is a wide range of intermediate manufacturing models. The scale-out manufacturing model supports the move towards de-centralized manufacturing, which helps to de-risk the manufacturing process against location-specific risk factors such as natural disaster, contamination and supply shortages. However, unless automation strategies are employed, the multiplicity of manufacturing locations dictates the need to train a large number of personnel trained to the same high level of competency [30].
Closed systems
Closed systems for cell therapy manufacturing refers to systems that maintain an aseptic environment during the cell culture process. These systems have an enclosed internal compartment where no contaminants can cross the barrier from outside to insider and vice versa, so that once the internal compartment has been sterilized, it remains sterile. From the regulatory point of view, closed systems contribute to Quality by Design (QbD). QbD is a regulatory expectation and an approach to manufacturing that is based primarily on risk assessment and management, as well as the implementation of monitoring and control processes [24]. Closed systems limit exposure to contaminants such as particles and microorganisms during the manufacturing process, maintaining aseptic conditions. Since CTP cannot be terminally sterilized, this is crucial throughout the manufacturing process. Thus, closed systems ensure CTP safety by maintaining the culture environment and is therefore aligned to the risk management and control aspects of the QbD approach.
Despite the benefits of keeping systems closed, it is necessary to perform product sampling to ensure product quality. Many protocols also involve addition of ancillary materials to maintain the cell culture or drive the selection of cell population. These ancillary materials are mostly biological molecules such as cytokines and growth factors that degrades at incubation temperature and thus have to be prepared and added to the culture during expansion. Thus, various strategies have been devised for closed system to accept ancillary material and to enable sampling during culture. The most common strategy is simply to open the closed system within a Class 100 cleanroom environment or a Biosafety Cabinet (BSC) that maintains the aseptic condition. While it is not ideal and may seem redundant to have a BSC next to a closed system, considering the high-value samples in CTPs, it would still be wise for manufacturers to equip facilities with BSCs as a failsafe measure. A few systems based on robotic arms enclosed within large isolators have portals where packages containing ancillary material are sterilized, typically by hydrogen peroxide [31], before they are transferred into the sterile internal compartment. Others such as the NANT 001 System (VivaBioCell S.p.A., UD, Italy) make use of a manifold designed with single-use aseptic connectors that can accept ancillary materials. These connectors are discussed in greater details under challenges in attaining full automation.
Single-use systems
A single-use system (SUS) refers to a system where the components in contact with the product are disposed after each use. In CTP manufacturing, SUS is typically the cell culturing surfaces such as the culture vessels and connected tubing. They may either be standalone vessels such as the Nunc™ Cell Factory™ System (Thermo Fisher Scientific Inc., Waltham, MA, USA), the Mobius® 3L Single-use Bioreactor (Merck KGaA, Darmstadt, Germany), and the VacciXcell™ Hybrid Bioreactor, or a component within and supported by a bigger closed system such as the hollow fibre bioreactor of the Quantum® Cell Expansion System and the CliniMACS Prodigy® Tubing Sets (Miltenyi Biotec GmbH, Bergisch Gladbach, Germany). For CTP manufacturing, automated systems tend to include some level of monitoring (see next section on on-line monitoring), which are performed by expensive equipment and sensors. Thus, where systems are automated, it is typical that the culture vessel is a single-use component aseptically connected to other persistent components such as the monitoring equipment.
All CTP manufacturing systems in the market are SUS as it eliminates concerns about cross-contamination arising from incomplete or inappropriate sterilization due to human error. Sterilization protocols of SUSs are required to undergo validation process in accordance with regulatory guidelines such as the USP Sterility Tests [32]. Thus, each culture starts with a high level of assurance that the vessel used is sterile so that it is unlikely a culture is discarded due to contamination with microorganisms or cross-contamination with cells from a previous culture. A failed culture, especially for autologous CTP, is not only costly, but may result in the patient missing the time window for effective treatment. Furthermore, assuming the unit cost of SUS will decrease as processes are standardized, and SUS may provide cost-savings in the long run.
Currently, issues with scaling up using SUS, such as the potential presence of leachables and extractables resulting from the polymer process during SUS production [33], is unclear. Recognizing the need for greater clarity in the regulatory requirements, new draft guidance such as the USP Plastic Components and Systems Used to Manufacture Pharmaceutical Drug Products [34] and USP Polymeric Components And Systems Used In The Manufacturing Of Pharmaceutical And Biopharmaceutical Drug Products [35], has been published and are being integrated into formal guidance. It is expected that with these new guidance and future refinements, SUS will be developed and certified to meet these safety requirements.
Monitoring
Monitoring of the cell culture is integral to its success. Several different aspects of the culture are commonly monitored: biological indications of cells’ health such as cell count, biomass and cell morphology; levels of physiochemical molecules such as pH and dissolved oxygen (DO); levels of chemicals such as glucose and lactate; presence or rate of biochemical processes within the cell [36,37]. In more specific bioprocesses, specialised sensors detect the presence of important biochemical processes such as the glycosylation of proteins [38].
Automated systems reduce the need for human handling of the culture, but since they tend to also be closed, access for monitoring using the conventional method of sampling and imaging is limited. This s driving the development of monitoring systems that are integrated into the automated systems to track culture parameters. In accordance with FDA’s quality management system guidelines, especially the PAT framework [25], the biopharmaceutical industry has committed increasing efforts in developing and adopting analytical tools for the on-line monitoring of bioprocesses. The PAT framework encompasses process understanding and quality monitoring to implement risk-based decision-making and to achieve the target quality. In the case of CTP manufacturing, real-time monitoring and analysis of key physical, chemical and biological variables is in line with PAT guidelines as it facilitates early fault detection and optimization of productivity, which consequently ensures final product quality.
The use of electrochemical sensors to monitor temperature, pressure, pH, dissolved gases, fluidic parameters and biomass is broadly employed in more matured systems with culture areas of larger than 1m2 and volumes of up to several hundred litres. These systems include the Quantum® Cell Expansion System, Xuri Cell Expansion System (GE Healthcare Life Sciences, Buckinghamshire, UK), TideCell® High Density Bioreactor System and VacciXcell™ Hybrid Bioreactor (Esco Micro Pte. Ltd., Singapore), as well as Xpansion® Multiplate Bioreactor System (Pall Life Sciences, Portsmouth, UK). For smaller scale cell expansions systems with cell culture areas of less than 0.1m2 and volumes of under 1L, it is also common to find imaging modalities that automatically perform a raster scan across the cell culture area, primarily to infer cell health from cell morphology and size. These systems include the NANT 001 and IncuCyte (Essen BioScience, Inc., Ann Arbor, MI, USA).
There are also systems that position themselves as add-ons, such as the iLine series by Ovizio (Ovizio Imaging Systems, Brussels, Belgium), CytoSMART by Lonza (CytoSMART Technologies B.V., Eindhoven, The Netherlands), and Real-time Cultured Cell Monitoring System by ASTEC (ASTEC CO., Ltd., Fukuoka, Japan). These systems simply add monitoring capabilities to existing cell expansion platforms and vessels and their monitoring capabilities are summarized in Table 2. They are useful in small-scale manual process development, where culture vessels can be brought to the monitoring systems at regular intervals or placed together with the monitoring systems in the incubator and monitored remotely. For full-scale production, they have to work in conjunction with other mechanical and fluid control systems to automate the process. To do so, they either adopt a non-contact approach of imaging through the culture vessel or a sterile closed-loop sampling of cells from the culture vessel.
Monitoring techniques and tools for bioprocesses have been outlined and reviewed in many review articles over the past decade [36,37,39–44]. The bioprocesses surveyed in these articles are mostly the production of proteins and protein-derivatives by microbial or mammalian cells, and have in recent years expanded to also encompass cell therapy products [41]. In agreement with these reviews, we observed in our survey of commercially available automated cell expansion systems, listed in Table 1, that there is a clear lack of adoption of these promising contemporary techniques. In later section on challenges of direct cell monitoring, we will explore technologies that need to be developed for more widespread adoption of such monitoring modules.
Table 2. Summary of ‘add-on’ monitoring systems. | ||||
---|---|---|---|---|
Company | Product | Application | Key technology | Monitoring Method |
Ovizio | iLine F | Adaptable to most stirred tank bioreactors | Differential digital holographic microscopy | Continuous closed-loop circulation of culture volume in stirred tank bioreactor; Cells captured under differential digital holographic microscopy - discerns between live and dead cells based on 3D morphology (label-free) |
Ovizio | iLine M | Usable with Corning® HYPERFlask®, Corning® CellSTACK® and NUNC™ Cell Factory™ Systems | Differential digital holographic microscopy | Multi-layer culture vessel is automatically scanned vertically (fixed x-y position) under microscope objective; Cells captured under differential digital holographic microscopy - discerns between live and dead cells based on 3D morphology (label-free) |
Ovizio | iLine S | Customized for Pall Life Science’s Xpansion Multiplate Bioreactor (up to 10 plates in monitoring) | Differential digital holographic microscopy | Multi-layer culture vessel is automatically scanned vertically (fixed window positions) under microscope objective; |
Lonza | CytoSMART™ System | Supports vessels of up to W133 x D90 x 100 mm | Image processing with wireless and cloud connectivity | Automated image processing of images captured at fixed point under microscope objective |
ASTEC | Real-Time Cultured Cell Monitoring System | Support vessels that can sit within a W350 x D350 x H11 mm space | Microscopy system that sits within incubator | Manual counting from images/videos |
Essen BioScience | IncuCyte® | Standard cell culture vessels and well plates; option to create custom vessel holders | Microscopy system that sits within incubator | Automated image processing of images captured through microscope objective across multiple vessels at programme positions |
Challenges
As more CTPs make it to market or progress into the final phases of clinical trials, the demand for better and more scalable manufacturing methods is increasing. The ideal state for CTP manufacturing is full automation, starting from collection of patient’s sample to infusion. Automation will help to ease the bottleneck of inadequately skilled labor, but it faces many unique challenges as a result of the complex workflow of CTP manufacturing, compounded with unclear regulatory requirements. While many bioprocesses have been successfully automated, the manufacturing challenges for CTP manufacturing and conventional bioprocessing such as protein and protein-derivative production differ significantly. For bioprocesses involving cells, the starting cell population is typically established cell lines with known characteristics and the bioprocesses are well studied. The opposite is true for CTP manufacturing as CTP is derived from primary cells from donors or patients. Cell-based products also do not permit terminal sterilization and filtration, thus requiring stringent manufacturing controls. In this section, we highlight some of the more pressing challenges impeding the automation efforts.
Aseptic connection
Aseptic connections are crucial in maintaining the sterility of CTPs. This is especially important during transfers between steps in CTP manufacturing. Current cGMP manufacturing turns to tube welding to create tube-to-tube connections. According to the guidance published by FDA [22], tube welding is regarded as an aseptic procedure if the weld integrity is tested to be satisfactory. However, tube welding can only be performed by trained personnel to ensure the quality of the tube weld. It is ineffective when tubing of different sizes and wall thicknesses are present, and adds significant operator time as each weld takes several seconds to complete [45].
An alternative to tube welding is manifolds designed with a pre-designed number of branching tubing with single-use aseptic connectors. Examples of aseptic connectors are the Kleenpak II Sterile Connector (Pall Corporation, Port Washington, NY, USA), Lynx® S2S connectors (Merck KGaA, Darmstadt, Germany) and genderless AseptiQuik connectors (CPC, St Paul, MN, USA). Aseptic connectors enable two sterile compartments to be connected in a non-sterile environment aseptically so that sterility is maintained after connection. To disconnect, the tubing is sealed off with a tube sealer, which contains a heating element that melts and seals across the tubing. While this reduces the need for keeping the entire system in an aseptic environment, it is limited to the pre-designed number of branched tubing. Furthermore, as the number of connections increases, the manifold quickly becomes massive and clumsy. Thus, single-use aseptic connectors are not scalable solutions.
The more scalable solution is a sterile reconnect that can maintain sterility over multiple disconnections and reconnections. The only product in the biotechnology industry that meets this criterion is the Lynx® CDR Sterile Connectors (Merck KGaA, Darmstadt, Germany). Unfortunately, the complexity of the mechanisms necessary to keep the internal environment sterile limits the application of this connector to large-scale bioreactors. The smallest hose barb size is 3/8 inch and the connector itself is larger than palm-size. While it may be useful in large-scale biopharmaceutical production lines, it is not suitable for CTP manufacturing as the initial culture volume tends to be small and many of the steps involve small volume transfer of expensive ancillary materials.
The challenge in creating suitable sterile reconnects for CTP manufacturing is associated with the difficulty of creating a sterile, drip-free mechanism designed for small volume transfer. In single-use sterile connector, each end of the tubing has a connector that are connected to allow sterile liquid flow. Each connector has a barrier, usually in the form of a membrane, that separated the internal sterile compartment from the external environment. This barrier is irreversibly broken upon connection as the barrier is inevitably exposed to the external environment. For example, the genderless AseptiQuik sterile connector features a flexible membrane that is bonded to a gasket around the opening of the tubing. To create a sterile connection, two connectors are connected tightly together via snap fits, compressing both the gaskets with two membranes in between them. This establishes a tight seal even as the membranes are pulled out to complete the connection. While the use of the flexible membranes is ingeniously simple and elegant, the initial bond between membrane and gasket cannot be recreated upon disconnect. To create a sterile reconnecting, the barrier thus has to be a rigid construct that is cleverly enclosed to prevent exposure, but such a design would be bulky and adds complexity.
In addition, to achieve sterile disconnection in a drip-free manner, a valve or barrier is required to separate the fluid into two bodies and prevent further fluid flow or transfer. In microfluidics, surface tension and hydrophobicity effects are dominant, and simple bite valves or duckbill valves are effective at separating and stopping flow. In larger scale bioprocesses, complex mechanical valves can be engineered. However, in CTP manufacturing, the internal tubing diameters, typically 1/8 to 1/4 inches, are too big for microfluidics designs to be effective and too small for common mechanical solutions to be implemented. Thus, clever design, coupled with the use of appropriate materials, and precision engineering are necessary to achieve sterile reconnects in CTP manufacturing.
There is no specific regulations or international standards for characterising or validating the aseptic quality of the aseptic connectors. Aseptic connectors are tested by the manufacturers to be leak-proof and to prevent contamination under certain conditions, but these tests are not standardised tests. It may be beneficial to develop a separate set of international standards to provide greater clarity for component manufacturers. In a similar fashion to the assurance conferred by the USP Class VI certification to the biocompatibility of polymeric materials, an international standard defining specific test conditions for aseptic connectors remove ambiguity for the conditions in which these aseptic connectors are validated for. For end users, this provides assurance. For component manufacturers, this clarifies the requirements and avoids over- and under- engineering of the aseptic mechanism.
On-line monitoring
Monitoring methods are categorised as on-line, at-line or off-line monitoring. In the context of cell manufacturing, on-line monitoring can be contact-based or non-contact modalities that are integrated into the cell expansion system to provide real-time information of the cells’ environment. Off-line or at-line monitoring are facilitated by sampling a small volume of the cell culture for further analysis with equipment away from the cell culture. Current monitoring methods in CTP manufacturing include: on-line monitoring of cell morphology using imaging modalities that are either integrated into or external to the system; on-line monitoring of the cells’ environment using integrated physical sensors; as well as off-line or at-line monitoring of the cells’ environment by performing quantitative measurements such as biochemical assays, high performance liquid chromatography, mass spectrometry, cell count and cell imaging on cell samples. While integrated pH, dissolved oxygen (DO) and temperature sensors are commonplace and seen in many systems listed in Table 1, other key molecules are still measured using off-line biochemical assays. In general, sampling is not ideal as it typically incurs a time delay before analysis and corrective action can be performed, results in wastage of the cell culture, and increases the risk of contamination. Unfortunately, designing an aseptic sampling device faces the same challenges as designing an aseptic connection.
Thus, integrated sensors for on-line monitoring are generally preferred. They provide real-time data without wastage and disturbing the cell culture. However, most integrated sensors can only perform bulk measurements of the cells’ environment and these are indirect measurements that often rely on comparison to an earlier time point to infer the current cell culture parameters. Consequently, calibration has to be performed whenever there are changes to the cell culture such as changes to cell type, seeding concentration and nutrient concentration. On the other hand, integrated imaging modalities offers direct measurement of cells through their morphology, but suffers from low throughput as the amount of cells per measurement is restricted to the cells found within the field of view (FOV) of the imaging apparatus, which is typically a millimeter-sized area. Conventional approaches either flow the cells through a flow cytometer or scan the cell culture area with an imaging apparatus. Such approaches are however impractical when scaling-up. Image-based measurements are nevertheless still the preferred method by biologist and medical professionals, as they are accustomed to characterizing cells based on morphological features. It would thus be a challenge in itself to convince stakeholders of these backgrounds to trust non-imaging techniques for cell-specific analysis.
Optical spectroscopy and chemometrics are emerging as new and potentially scalable technologies for cell monitoring and are highlighted in many review articles on bioprocess monitoring [36,37,39-44]. Optical spectroscopy measures changes in the light properties across the electromagnetic spectrum after interaction with a material to infer material properties. It can measure biological variables like turbidity, biomass and cell viability. Chemometrics refers to data analytics based on mathematical models that allow for the understanding and correlation of data to process variables. Chemometrics leverages the advancing computational capabilities to support more frequent and comprehensive on-line monitoring, where large data sets improve noise removal and allow meaningful inferences even from data that are measured by simpler and less costly hardware. Notably, optical spectroscopy and chemometrics are rarely employed in practice as most of them are still prevalently developed for indirect cell measurements of the cells’ environment which are already achieved reliably and at a lower cost with conventional physical sensors and electrodes. In contrast to the present bioprocess context where cells release the therapeutic products into the cell culture, cells are the target product in CTP manufacturing. Thus, optical spectroscopic techniques have potential advantages over contact-based sensors as they are capable of probing a wide range of chemical and biological variables within the cells that have molecular specificities via fluorescence [40] and Raman [43,46]. The technical difficulties of implementing optical spectroscopy techniques, such as noise from the culture media and high equipment cost, may be offset by the data-driven approach in chemometrics. With a large amount of data collected, chemometrics may be able to extract useful information from weaker and noisier data obtained with simpler and cheaper optical spectroscopy tools.
On the other hand, handling and interpreting such massive amounts of data might in itself be a challenge, particularly in real-time monitoring. This is especially true in cell measurements, where multi-dimensional data may be captured simultaneously. In chemometrics, multivariate analysis approaches such as principal component analysis (PCA) and artificial neural networks (ANN) have been demonstrated to be capable of extracting key information and patterns. These approaches, however, require substantial amounts of known data to first be acquired for modelling before they can actually be applied in the manufacturing context. Nevertheless, the modelling, optimization and calibration of the monitoring module is commonly done during the development phase of the module. Earlier adoption of the module provides more time and data for the development and refinement of the model.
Logistics for needle-to-needle traceability
In compliance with cGMP, all pharmaceutical drugs and biologics have to be tracked along the supply chain. Any devices, including automated devices, introduced into the manufacturing process must be able to be maintain the traceability of all reagents and materials. This establishes a chain of identity, where any material used and made in the process are checked and identified, and a chain of custody where every change of custody is documented. Full traceability in CTP manufacturing starts from the extraction of donor or patient material at bedside, and ends at the infusion of the final CTP to the patient at bedside. The tracking of this unbroken chain of events is aptly termed as needle-to-needle traceability [47].
To achieve needle-to-needle traceability, tracking has to be done across many clinical and non-clinical sites and involve many stakeholders. Labels maintain the chain of identity during transportation and every site that handles the material should be able to print quality labels on the go. Anecdotally, many labels tend to fade or peel off along the supply chain as the sample is exposed to handling and extreme temperatures in storage. The loss of labels can cause delayed and even rejection of product due to safety concerns.
Current management systems have been argued as inadequate to manage the complex supply chain for CTP manufacturing, and cloud-base Cell Orchestration Platforms (COPs) are developed to complement existing patient management and quality management systems [47]. The COP pulls data into a workflow customized for each CTP and tracks every event and change of custody within a single interface. It acts as a layer above these systems and also fills in any process gaps that are not captured within existing systems [47]. Thus, for a COP to be effective, it has to be compatible across all systems currently used by all stakeholders, and also accommodate the multitude of manufacturing processes for different CTPs. The development of such a universal platform requires concerted efforts by influential stakeholders to either adopt existing standards or establish new standards for the communication protocol between systems.
Cold chain
As CTP manufacturing scales, the need for cold chain logistics for safe and timely delivery of CTP becomes apparent. The cold chain refers to the uninterrupted chain of temperature-controlled transportation of a material. As cells require strict climate control to thrive, they are typically cryopreserved for transport between locations. If the CTP is manufactured at a location away from the clinic where patient or donor sample is collected or infused, the cold chain will then be necessary to transport the cell sample to manufacturing sites, between manufacturing sites, and/or to final clinical site for administration. This is currently the case for most CTP manufacturing. However, while the cold chain is already established in many food and drug industries, CTP manufacturing presents additional challenges to the traditional cold chain logistics [48]. Patients may be too frail and cause delays to the original delivery schedule, or may develop new complications that postpone the treatment. Manufacturing time for autologous CTP manufacturing depends heavily on the quality of the patient’s sample and delivery plans may only be confirmed with a very short notice. Compounding these delays and short notices, the final CTP cannot survive long periods of cryopreservation and must be delivered promptly. With autologous or small-scale manufacturing, the CTP is targeted only for single patient, which further fragments the cold chain and adds to the logistical challenges [49]. These constraints require the cold chain provider to understand and appreciate the scheduling and coordination challenges inherent to the manufacture process and to have the resources to accommodate flexibility in the service agreement while maintaining a high quality of service [50]. The challenges and complexity of the cold chain is eloquently described here with illustrations and the introduction of a four-part planning process [51]. On the other hand, if CTP is fully decentralised and is manufactured at bedside in the clinic, the cold chain is not necessary. Automated cell expansion systems could conceivably de-centralize CTP manufacturing [52], eliminating the need for extensive cold chain logistics associated with the preservation and transportation of CTP.
While fully closed and automated systems are being developed, Asymptote (Asymptote Ltd, Cambridge, England, UK) has developed GMP freezing and thawing systems to improve the robustness of the cold chain against human error. The current freezing and thawing processes performed in labs involve the use of water baths that pose contamination risks and the cells are susceptible to temperature fluctuation during handling. The GMP freezing and thawing systems developed by Asymptote aims to control the freezing and thawing rates so that these steps do not affect product quality. A closed GMP device for controlled thawing may also enable the clinic to rehabilitate CTP just prior to infusion to allow the CTP to recover [50].
Regulatory framework
As the cell therapy industry is very new with limited clinical data, very little in current guidelines are specific to CTP manufacturing. Focusing on the US Pharmacopeial Convention, which publishes the US Pharmacopeia (USP), new chapters and updated chapters are added every year in a new version of the USP to reflect the changing regulatory landscape for therapeutic products. As early as in year 2000, the USP included a new chapter USP Cellular and Tissue-based Products [1] to discuss topics on the manufacturing and testing of CTP [53]. However, 17 years later, in the USP41-NF36 publication, this chapter still does not explicitly indicate the release criteria for CTP. While it states specific thresholds for sterility, mycoplasma and endotoxin levels, all the other requirements such as purity, potency, identity and dose are still left as ‘product specific’.
The industry has looked to using other USP chapters for pharmaceuticals to guide their CTP development. For example, guidelines on the thresholds for particulates of various sizes are documented only in USP Particulate Matter in Injections [54] and USP Subvisible Particulate Matter in Therapeutic Protein Injections [55], and they may not be suitable guidance. In CTP manufacturing, since the cells are the final therapeutic product, the exposure of the cells to particulates across the entire manufacturing timeframe may have a different impact than particulates that are present in pharmaceuticals. It was only recently in 2017, that two guidances, USP Plastic Components and Systems Used to Manufacture Pharmaceutical Drug Products [16] and USP Polymeric Components And Systems Used In The Manufacturing Of Pharmaceutical And Biopharmaceutical Drug Products [17], were drafted to guide the evaluation of polymeric equipment such as culture vessels for cytotoxicity. These still remain as draft guidance and are delayed in the incorporation into the official USP publication. Another relevant chapter USP Plastic Packaging Systems and their Materials of Construction [56] is split into two chapters Plastic Materials of Construction [57] and USP Plastic Packaging Systems for Pharmaceutical Use [58] to more comprehensively cover chemical risk assessment such as leachables and extractables.
It is challenging to develop explicit guidelines for CTP mainly because of the lack of scientific evidence specific to CTP and the large variety of CTPs in clinical trials. Thus, the current regulatory landscape for CTP manufacturing exhibits a heavy dependence on good science, affirmative pre-clinical trials or early-phase clinical trial results, verified safety and quality profiles, and thorough documentation that demonstrates a validated and controlled manufacturing process. More data is needed to dissect and scrutinize the risks and benefits of the various aspects of CTP and CTP manufacturing. Given the large variations in the quality of raw materials and the diversity of CTPs in the development pipeline, it will take concerted international effort to delineate the appropriate regulations. Various regulatory bodies such as the USP, the Pharmaceutical and Medical Device Agency (PDMA; Chiyoda-ku, Tokyo, Japan), the BioPhorum Operations Group (BPOG; BioPhorum Operations Group, Sheffield, UK), the BioProcess Systems Alliance (BPSA; Arlington, VA, USA) and the International Council for Harmonisation (ICH; Geneva, Switzerland) are leading efforts to develop harmonised standards specifically for CTPs and CTP manufacturing. Until then, developers seeking regulatory approval are relying on their own interpretation based on existing standards for pharmaceutical products. The ambiguity of the regulation can result in additional costs arising from either compliance to standards that are too stringent, or corrective measures as a result of compliance to inappropriate standards.
Outlook
Cell therapy is a promising field for treating diseases where traditional strategies have failed. Given the high dependence on skilled and expensive labor of current cell therapy product (CTP) manufacturing process, fully automated CTP manufacturing promises to reduce per treatment cost. This is key to bringing cell therapy to more patients, which in turn generates more data that feeds back into developing better CTP and regulatory guidelines. In this regulatory landscape, automation of the CTP manufacturing process is both a hurdle and an aid to CTP developers. Automation of a process requires the process to be established with known control parameters, without which, it is difficult to prove that the automated process is safe and equivalent to the manual open process. On the other hand, automation could provide the repeatability and on-line control that are lacking with manual processes, while at the same time lowering labor costs. In addition, the use of automated systems could simplify the documentation and traceability of the CTP manufacturing according to regulatory requirements. However, even as CTP manufacturing processes gain clarity and the industry gains experience and knowledge from on-going clinical trials, there are still many technological developments that need to happen to achieve full automation. The CTP manufacturing community needs to work together to overcome the various challenges highlighted in this review in order for cell therapy manufacturing to be fully automated.
Acknowledgements
This work is supported by the Agency of Science Technology and Research (A*STAR), Singapore.
financial & competing interests disclosure
The authors have no relevant financial involvement with an organization or entity with a financial interest in or financial conflict with the subject matter or materials discussed in the manuscript. This includes employment, consultancies, honoraria, stock options or ownership, expert testimony, grants or patents received or pending, or royalties. No writing assistance was utilized in the production of this manuscript.
References
1. United States Pharmacopeial Convention. Cellular and tissue-based products. Standard. Baltimore, MD, U.S.A.: United Book Press, Inc.; 2017. Report No.: USP41.
2. Davis AH. New Gene Therapy CMC Guidance from the FDA – A Breakthrough in Regulation or Something More Generic? Cell Gene Ther. Ins. 2018; 4(6): 647–52. CrossRef
3. Newick K, O’Brien S, Moon E, Albelda SM. CAR T Cell Therapy for Solid Tumors. Annu. Rev. Med. 2018; 68(1): 139–52. CrossRef
4. Sanganalmath SK, Bolli R. Cell therapy for heart failure: a comprehensive overview of experimental and clinical studies, current challenges, and future directions. Circ. Res. 2013; 113(6): 810–34. CrossRef
5. Piuzzi NS, Chahla J, Schrock JB et al. Evidence for the Use of Cell-Based Therapy for the Treatment of Osteonecrosis of the Femoral Head: A Systematic Review of the Literature. J. Arthroplasty 2017; 32(5): 1698–708. CrossRef
6. Ibrahim A, Cheng K, Marbán E. Exosomes as Critical Agents of Cardiac Regeneration Triggered by Cell Therapy. Stem Cell Rep. 2014; 2(5): 606–19. CrossRef
7. Phinney DG, Pittenger MF. Concise Review: MSC-Derived Exosomes for Cell-Free Therapy. Stem Cells 2018; 35(4): 851–8. CrossRef
8. Ginn SL, Amaya AK, Alexander IE, Edelstein M, Abedi MR. Gene therapy clinical trials worldwide to 2017: An update. J. Gene Med. 2018; 20(5): e3015. CrossRef
9. TechNavio. Global cell therapy market 2017-2021. Report. Research and Markets; 2017. Report No.: 4392588.
10. Phacilitate, Biotech and Money. Advanced therapies investment report 2017. Investment Report. Web: Phacilitate & Biotech and Money; 2017.
11. KYMRIAH (tisagenlecleucel) [Internet]. Web: US Food and Drug Administration; 2018: https://www.fda.gov/biologicsbloodvaccines/cellulargenetherapyproducts/approvedproducts/ucm573706.htm
12. YESCARTA (axicabtagene ciloleucel) [Internet]. Web: US Food and Drug Administration; 2018: https://www.fda.gov/BiologicsBloodVaccines/CellularGeneTherapyProducts/ApprovedProducts/ucm581222.htm
13. McDonald-Hyman C, Turka LA, Blazar BR. Advances and Challenges in Immunotherapy for Solid Organ and Bone Marrow Transplantation. Science Trans. Med. 2015; 7(280): 280rv2-. CrossRef
14. Yang Y, Jacoby E, Fry TJ. Challenges and opportunities of allogeneic donor-derived CAR T cells. Curr. Opin. Hematol. 2015; 22(6): 509–15. CrossRef
15. Huang R, Yao K, Sun A et al. Timing for intracoronary administration of bone marrow mononuclear cells after acute ST-elevation myocardial infarction: a pilot study. Stem Cell Res. Ther. 2015; 6(1): 112. CrossRef
16. Naseri MH, Madani H, Ahmadi Tafti SH et al. COMPARE CPM-RMI Trial: Intramyocardial Transplantation of Autologous Bone Marrow-Derived CD133(+) Cells and MNCs during CABG in Patients with Recent MI: A Phase II/III, Multicenter, Placebo-Controlled, Randomized, Double-Blind Clinical Trial. Cell J. 2017; 20(2): 267–77. CrossRef
17. Vormittag P, Gunn R, Ghorashian S, Veraitch FS. A guide to manufacturing CAR T cell therapies. Curr. Opin. Biotechnol. 2018; 53: 164–81. CrossRef
18. Bartunek J, Behfar A, Dolatabadi D et al. Cardiopoietic Stem Cell Therapy in Heart Failure: The C-CURE (Cardiopoietic stem Cell therapy in heart failURE) Multicenter Randomized Trial With Lineage-Specified Biologics. J. Am. Coll. Cardiol. 2013; 61(23): 2329–38. CrossRef
19. Brogdon J, June CH, Loew A, Maus M, Scholler J, inventors; Novartis AG, University of Pennsylvania, assignees. Treatment of cancer using humanized anti-cd19 chimeric antigen receptor. USA patent US20140271635A1. 2014.
20. Perez A, Sabatino M, Rosenberg SA, Restifo NP, inventors; NATIONAL CANCER INSTITUTE, Kite Pharma Inc, assignees. Methods of Preparing T Cells for T Cell Therapy. US patent US20170136063A1. 2017.
21. Lopes AG, Sinclair A, Frohlich B. Cost Analysis of Cell Therapy Manufacture: Autologous Cell Therapies, Part 2. BioProcess Int. 2018; 16(4): 12–9.
22. US Food and Drug Administration. Guidance for Industry: Use of Sterile Connecting Devices in Blood Bank Practices. Guidance. MD, U.S.A.: U.S. Food and Drug Administration; 2000 November.
23. Kowalski S, Kranjac D. Interview: Innovative technologies for CAR-T manufacturing: the key to commercialization. Cell Gene Ther. Ins. 2018; 4(4): 337–44. CrossRef
24. US Food and Drug Administration. Guidance for Industry: Quality Systems Approach to Pharmaceutical CGMP Regulations. Guidance. MD, USA: US Food and Drug Administration; 2006 September.
25. US Food and Drug Administration. Guidance for Industry: PAT — A Framework for Innovative Pharmaceutical Development, Manufacturing, and Quality Assurance. Guidance. MD, USA: US Food and Drug Administration; 2004 September.
26. Novartis Has Production Hiccup for Kymriah CAR-T Therapy [Internet]. Web: BioPharm International; 2018: http://www.biopharminternational.com/novartis-has-production-hiccup-kymriah-car-t-therapy-0. <
27. Bhansali S, Dutta P, Yadav MK et al. Autologous bone marrow-derived mononuclear cells transplantation in type 2 diabetes mellitus: effect on β-cell function and insulin sensitivity. Diabetol. Metab. Syn. 2017; 9(1): 50. CrossRef
28. Liotta F, Annunziato F, Castellani S et al. Therapeutic Efficacy of Autologous Non-Mobilized Enriched Circulating Endothelial Progenitors in Patients With Critical Limb Ischemia- The SCELTA Trial. Circ J. 2018; 82(6): 1688–98. CrossRef
29. Kaiser AD, Assenmacher M, Schröder B et al. Towards a commercial process for the manufacture of genetically modified T cells for therapy. Cancer Gene Ther. 2014; 22(2): 72–8. CrossRef
30. Goodman S. Interview: Opportunities and Challenges for Decentralizing Cell and Gene Therapy Manufacturing. Cell Gene Ther. Ins. 2018; 4(5): 485–94. CrossRef
31. Kino-oka M, Mizutani M. Comprehensive cell manufacturing system based on flexible modular platform 85. Scale-up and Manufacturing of Cell-based Therapies V; SD, USA: ECI Symposium Series; 2017.
32. United States Pharmacopeial Convention. Sterility tests. Standard. Baltimore, MD, U.S.A.: United Book Press, Inc.; 2017. Report No.: USP41.
33. Single-use systems for biotechnology products [Internet]. Web: European Pharmaceutical Review; 2018: https://www.europeanpharmaceuticalreview.com/article/50692/single-use-systems-biotechnology-products/. CrossRef
34. United States Pharmacopeial Convention. Plastic components and systems used to manufacture pharmaceutical drug products. Standard. Baltimore, MD, U.S.A.: United Book Press, Inc.; 2017. Report No.: USP41. CrossRef
35. United States Pharmacopeial Convention. Polymeric components and systems used in the manufacturing of pharmaceutical and biopharmaceutical drug products. Standard. Baltimore, MD, U.S.A.: United Book Press, Inc.; 2017. Report No.: USP41.
36. Randek J, Mandenius CF. On-line soft sensing in upstream bioprocessing. Crit. Rev. Biotechnol. 2018; 38(1): 106–21. CrossRef
37. Clementschitsch F, Bayer K. Improvement of bioprocess monitoring: development of novel concepts. Microbial Cell Factories. 2006; 5: 19. CrossRef
38. Tharmalingam T, Wu C, Callahan S, Goudar C,T. A framework for real-time glycosylation monitoring (RT-GM) in mammalian cell culture. Biotechnol. Bioeng. 2018; 112(6): 1146–54. CrossRef
39. Lourenco ND, Lopes JA, Almeida CF, Sarraguca MC, Pinheiro HM. Bioreactor monitoring with spectroscopy and chemometrics: a review. Anal. Bioanal. Chem. 2012; 404(4): 1211–37. CrossRef
40. Faassen SM, Hitzmann B. Fluorescence Spectroscopy and Chemometric Modeling for Bioprocess Monitoring. Sensors (Basel, Switzerland). 2015; 15(5): 10271–91. CrossRef
41. Mandenius C, Robert G. Mini-review: soft sensors as means for PAT in the manufacture of bio-therapeutics. J. Chem. Technol. Biotechnol. 2015; 90(2): 215–27. CrossRef
42. Classen J, Aupert F, Reardon KF, Solle D, Scheper T. Spectroscopic sensors for in-line bioprocess monitoring in research and pharmaceutical industrial application. Anal. Bioanal. Chem. 2017; 409(3): 651–66. CrossRef
43. Buckley K, Ryder AG. Applications of Raman Spectroscopy in Biopharmaceutical Manufacturing: A Short Review. Appl. Spectrosc. 2017; 71(6): 1085–116. CrossRef
44. Teixeira AP, Oliveira R, Alves PM, Carrondo MJ. Advances in on-line monitoring and control of mammalian cell cultures: Supporting the PAT initiative. Biotechnol. Adv. 2009; 27(6): 726–32. CrossRef
45. Pendlebury D. Cell & gene therapies: A guide to single-use connections – 10 transferable lessons from the bioprocessing industry. Bioprocess Online. 2018.
46. Baradez M, Biziato D, Hassan E, Marshall D. Application of Raman Spectroscopy and Univariate Modelling As a Process Analytical Technology for Cell Therapy Bioprocessing. Front. Med. 2018; 5: 47. CrossRef
47. Lamb M, Margolin RE, Vitale J. Expert insight: Personalized supply chains for cell therapies. Cell Gene Ther. Ins. 2017; 3(10): 815–33. CrossRef
48. Shanley A. Preparing an Approach to Cold Chain for Cell- and Tissue-Based Therapies. Pharm. Technol. 2017; Suppl. 1: 8–12.
49. Gene and Cell Therapy: The top 5 challenges for cold chain logistics [Internet]. Germany: Nelumbox; 2017: https://nelumbox.com/2017/10/gene-and-cell-therapy-the-big-5-challenges-for-cold-chain-logistics/.
50. Coopman K. Interview: Logistical considerations of the cell therapy supply chain at the point of care. Cell Gene Ther. Ins. 2018; 4(5): 501–8. CrossRef
51. World Courier. Tomorrow’s Medicine: Curing One Patient at a Time Global Logistics Strategies for Cell and Gene Therapies. AmerisourceBergen Corporation; 2017.
52. Harrison RP, Ruck S, Medcalf N, Rafiq QA. Decentralized manufacturing of cell and gene therapies: Overcoming challenges and identifying opportunities. Cytotherapy 2017; 19(10): 1140–51. CrossRef
53. Seaver S. A new United States Pharmacopeia (USP) Chapter 1046: cell and gene therapy products. Cytotherapy 2000; 2(1): 45–9. CrossRef
54. United States Pharmacopeial Convention. Particulate Matter in Injections. Standard. Baltimore, MD, USA: United Book Press, Inc.; 2017. Report No.: USP41.
55. United States Pharmacopeial Convention. Subvisible Particulate Matter in Therapeutic Protein Injections. Standard. Baltimore, MD, USA: United Book Press, Inc.; 2017. Report No.: USP41.
56. United States Pharmacopeial Convention. Plastic Packaging Systems and their Materials of Construction. Standard. Baltimore, MD, USA: United Book Press, Inc.; 2017. Report No.: USP41.
57. United States Pharmacopeial Convention. Plastic Materials of Construction Standard. Baltimore, MD, USA: United Book Press, Inc.; 2017. Report No.: USP41.
58. United States Pharmacopeial Convention. Plastic Packaging Systems for Pharmaceutical Use. Standard. Baltimore, MD, USA: United Book Press, Inc.; 2017. Report No.: USP41.
Affiliations
Ying Ying Wu1, Derrick Yong2 & May Win Naing1*
1Bio-Manufacturing Programme, Singapore Institute of Manufacturing Technology, A*STAR, 2 Fusionopolis Way, Innovis #08-04, Singapore
2Precision Measurements Group, Singapore Institute of Manufacturing Technology, A*STAR, 2 Fusionopolis Way, Innovis #08-04, Singapore