Changing the ‘Art’ of Growing Cells into an Industrialized Platform for Commercial-Scale Manufacturing
Cell Gene Therapy Insights 2018; 4(8), 805-814.
10.18609/cgti.2018.082
For the last few years, the cell therapy industry has evolved from a ‘research’ state of mind to a commercialization focus. The recent approvals for Kite, Novartis and Tigenix have boosted the evolutionary process and changed the focus from ‘how to bring the product to approval?’ to ‘what will happen afterwards?’. Based on the proof that cell therapies do work and hold the potential to change the way medicine is practiced, the cell therapy industry is now mature enough to start facing the gaps in translating the manufacturing lines into industrialized platforms.
Process gaps that make todays manufacturing an art
The statement that the process affects the product is true for almost every manufacturing line. However, for cell therapies this statement has a higher level of meaning. Since cells are living products, they can interact and react to changes during the process [2]. Keeping the product under control during the process actually means keeping the process controlled from the isolation step and up to the patient’s body. However, current technologies hold many gaps for every process step. Most of these gaps are around manual operation, poor process control, open manipulations and scale. Early-stage cell therapy manufacturing is often done, for good reasons, on platforms that are not suitable for industrialization. Most of these platforms are standard 2D technologies (T-flasks, cell factories, cell stacks etc.). For the downstream steps, the challenges begin in product harvesting from the growth platform and continue up to the final packaging. During these steps, the cells are exposed to harsh conditions such as uncontrolled environment, shear forces and prolonged holding times that damage the cells. Finally, as more and more late-stage clinical experiments have been initiated over recent years, there has been an increase in understanding that the cell therapy process does not end in the final product packaging: in fact, there is a bridge between the manufacturing line and the patient’s bedside, and its building blocks are shipment, storage, thawing and administration. These steps in the cell therapy process are poorly controlled and different solutions must be developed in order to provide the highest quality product to patients.
The importance of process development in changing the art into an industry
In order to transform cell therapy to an industry and be able to supply the post-approval clinical demand, companies must have the following five aspects in their process:
- Good and potent cell source
- Closed manufacturing systems
- Reproducible process
- Ability to produce trillions of high-quality cells per year
- Low cost of goods
Good & potent cell source
Apart from the impact of the cells source on the product potency, other process-related parameters are entangled to the selection of the source. The cell source and its target product profile (TPP) have one of the biggest impacts on the design of the manufacturing line. The first step is to decide whether your TPP is autologous or allogeneic.
In autologous products, where cells are obtained from a donor and are aimed to treat only the donor himself, there is no need to scale up a batch more than the number of cells needed for treatment (hundreds of million cells). Therefore, the industrialization of autologous treatments is more focused on scaling facility capacities in order to handle multiple batches at a time in a closed and automated manner, and on cold chain control and capabilities. On the other hand, in allogeneic products there is no need to match between the donor and the patient – thus, in order to fully harness the product’s potential for commercialization, scale up of the process is needed in order to reach the point of producing millions of doses per batch.
Another aspect of cell source is the cells’ ability to proliferate and their limit of doublings. Different cell types have different proliferation capacity until they lose potency, reach senescence or mutate. In order to maximize the growth potential within the doubling limit window there is a need to dramatically increase the growth surface area – thus, scaled up systems is the only solution to take full advantage of allogeneic therapies within a reasonable infrastructure. It should also be remembered that raw material availability, sample collection complexity and donor health are directly related to the quality of the product at the end of the process.
A good example for all these considerations is Pluristem’s manufacturing process. Our products are placenta derived adherent stromal cells. Placenta is considered as medical waste, but on the other hand it is a rich and diverse source with plenty of highly potent cells, it comes from young donors (high starting material quality), it is unlimited, easy to collect (no manipulation on the donor), ethically accepted and most importantly, placenta-derived cell therapies are allogeneic in nature and therefore scaling up will unleash the product potential. Having all these benefits together helped us design a process were the same cell source can serve as a platform for multiple products and is not considered as a limiting factor for large-scale manufacturing.
Closed manufacturing systems, reproducible process & ability to produce trillions of high-quality cells per year
The main goal of process development is to transform ideas into products that could be manufactured in an industrialized process while maintaining product specification. In the end, the goal is to build a system that will be able to provide the same product, in the highest quality, the lowest risk and in the amount needed for the marketing stage. To increase the chance for succeeding in getting a cell therapy to market, it is of utmost importance to consider multiple aspects of process development from the earliest possible stage. All elements of the process, from cell isolation and proliferation to removal of impurities, final formulation, packaging, and storage, should be taken into consideration, from technical, regulatory and commercial aspects.
Process development challenges inherent in all cell therapies include the optimization of cell culture methods, scale-up and scale-out, and removal of impurities. The best way to reach a goal is to first understand what is the target, and then to draw a bridge from the end back to the beginning and start building it step by step [3]. By applying tools such as quality by design (QBD), process gaps analysis, trends analysis and continuous improvement, a successful translation could occur (Figure 1).
From the technology point of view, industrializing the process involves the implementation of new technologies that allow process automation, control and closed manufacturing systems. Having these capabilities in the manufacturing process allows the implementation of process analytical technologies (increasing control), reduction of the human factors (and errors) with the potential to harm the product and increase the batch-to-batch consistency. Finally, the ability to scale up and automate the process leads to reduction in cost of goods due to the increase in doses per batch, reduction of manpower and lower infrastructure needed. All these changes when implemented will change the art of growing cells into an industrialized process.
Process development evolution
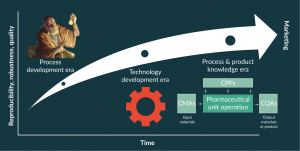
Figure 2. The three eras of process development evolution: process development era, technology development era and process and product knowledge era.
Process development is an evolutionary process entangled with the understanding of product TPP, mode of action (MOA) and critical quality attributes (CQA). Translation of a manual processes to industrial grade processes may significantly alter cell properties (e.g., differentiation potential, immunosuppressive capacity) [5,6]. In order to protect the product specification, the main objective in process development is to learn how the process effects the product specifications and then apply control strategies on the critical process parameters (CPP). The CPP’s are process variables that influence the CQA.
There are three main stages in process development:
- Process development era
- Technology development era
- Process & product knowledge era
When all three development steps are done correctly, the product quality, reproducibility and robustness will increase (Figure 2).
Process development era
In the first steps of process development, experiments are performed in order to define the correct process steps needed to produce the desired product. The process is divided into units of operation, and a definition of inputs and outputs for each unit of operation is performed (Figure 3). In most cases during the process development era, the procedures are still manual and operated independently. However, applying well-defined batch records reduces the variation that originates from different operators. Once inputs and outputs are defined, it is very important to start collecting the data and performing process trend analysis. Special attention needs to be paid to changes in the outputs trends because these are signs of CPPs.
Technology development era
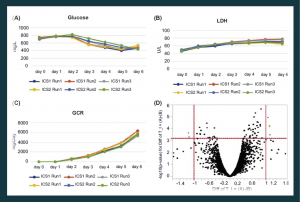
Figure 4. Bioreactors enable real-time monitoring of cell growth and more consistent products. Glucose (A), LDH levels (B) and glucose consumption rate (C) were monitored in six bioreactor runs originating from two placenta donors (designated ICS1 and ICS2). The results demonstrate a highly uniform cell manufacturing process. (D) A microarray-based gene expression analysis of nine bioreactor runs from three placenta donors showing very few genes (light gray dots, six genes) whose expression is altered in a statistically significant manner (two-fold difference in level, P < .05).
GCR: Glucose consumption rate; ICS: Intermediate cells stock; LDH: Lactate dehydrogenase.
Following the successful definition of the required units of operation, the right sequence of these units, and the inputs and outputs for each one, it is now feasible to start to close and automate the process. Since in most cases there is limited funding, a risk-based approach is a useful tool for the selection of where to begin this stage. For each unit of operation, an analysis of risk for contamination, complexity of operation, level of process control needed, the effect that the technology could have on the product at this step (i.e., shear forces) and many other considerations is performed. Based on that analysis, targeted projects are initiated to evaluate existing technologies, or need for development of new technologies, for each unit of operation.
The main challenges of this step relate to changes to the inputs defined in the process development era. Closing the process and incorporating automation changes the way some of the processes are performed and each change leaves a fingerprint on the product. For example, in most industrialized closed processes, pumps are used for the transfer of fluids. Pumps are a source of shear forces acting on the cells. It is known that shear forces can cause changes to cells ranging from differentiation to apoptosis [7–9] so it is important to learn the effect of these forces on the product in different units of operation.
Very early on in Pluristem’s process development lifecycle, we understood that using the standard 2D technology (cell factories/cell stacks) would not be sufficient once we are in the commercialization phase. This understanding led us to make two very important decisions: we would invest in technology before entering clinical phases in order to be commercialization-ready at every step; and we will manufacture our product in-house in order to fully understand and control the effects of the manufacturing process on the product. Based on these decisions, we implemented our unique bioreactor technology: the use of 3D bioreactors (as opposed to conventional 2D growth platforms) allows for increases in the number of cells to be grown in smaller physical areas, and with smaller volumes of costly reagents. This has the potential to enable mass production of cells in a cost-effective way and to make the cell therapy economically feasible. In addition, although they require higher initial capital investment, bioreactors enable automated monitoring and adjustments of physical and chemical factors that can affect cell growth, such as pH, dissolved oxygen (DO) and temperature.
Continuous monitoring and adjustment of these parameters using fed-batch and perfusion systems can reduce batch-to-batch variation to levels not achievable by traditional 2D platforms [10]. For example, Figure 4 illustrates various growth parameters of six bioreactors runs originating from two different placenta donors (designated as intermediate cell stock 1 (ICS1) and ICS2). It is evident that glucose (Figure 4A) and lactate dehydrogenase (LDH) (Figure 4B) levels, as well as glucose consumption rates (GCR) (Figure 4C), are highly uniform. This enables high batch-to-batch consistency and a robust final product, as evidenced by whole-genome expression analyses. For example, when nine bioreactor runs from three placenta donors were compared, only six genes were differentially expressed at the RNA level in a statistically significant way (Figure 4D) and none of these were expected to be involved in the cell’s MoA.
Process & product knowledge era
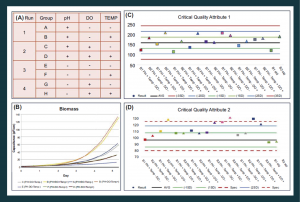
Figure 5. Bioreactor studies enable close monitoring of cell growth and allow a better understanding of the various parameters affecting the final cell therapy product. (A) Overview of layout of example bioreactor studies. (B) Biomass comparison between bioreactor runs indicated in (A). (C & D) Two critical quality attributes measured in bioreactor studies, to identify differences caused by pH, DO and temperature changes. DO: Dissolved oxygen.
Once the process has been defined and technology incorporated, the ability to ‘play’ with process inputs is at its highest level, and a true understanding of the effect of inputs on outputs can be achieved. In this step, a design of experiments (DOE) approach is advised for learning how multiple inputs affect the CQA in parallel. Furthermore, it is advisable to add another variable into the equation in order to achieve the highest level of control possible. The final variable is the effect of critical raw material attributes (CMA) on the process and product.
During the process and product knowledge step, the CPPs and CMAs are detected and by using the DOE approach, correct design spaces are implemented as a control strategy. For example, the use of 3D reactors and real-time monitoring of various parameters enable the study of how various process parameters affect cell growth and characteristics. The effects of three parameters on cell growth were tested (pH, DO and temperature), see Figure 5A. GCR (not shown) and biomass (Figure 5B) were monitored. The results indicate that higher pH and temperature enhance these parameters, as well as improve the harvest yield (not shown), with respect to not only biomass but also the ratio of live to dead cells. Two critical quality attributes routinely tested in QC were highly informative (Figure 5C & D): the first was similar in all tested bioreactors, whereas the second (CQA2) indicated that some process parameters raised values beyond the QC specifications. This illustrates the benefit of a well-controlled closed bioreactor system with respect to the control over the process, and the enablement of a deeper understanding of parameters affecting the cell therapy product.
Advancing through the process development evolution requires initial investment in the form of time and money. However, the course of increasing the process knowledge and understanding will lead to improved yields, higher process robustness and reduced cost of goods (Table 1).
Table 1. Process development steps effect on cost of goods. | |
---|---|
Effect | Impact on COG |
Understanding the MOA | Ability to identify alternative raw materials without risk of changing the product |
Ability to perform process upgrades without risk of changing the product | |
Technology and closed system | Reduce failure rate and product loss in the process |
Automation | Reduced amount of personal needed |
Understanding critical material attributes | Ability to control material cold chain |
Ability to identify alternative raw materials | |
Develop formulations that have the needed components in the needed concentrations | |
Process knowledge and control | Ability to define parameter ‘sweet spots’ that will increase yield |
TRANSLATIONAL INSIGHT
Cell therapy products hold great potential to change the way medicine is being practiced. However, there is an enormous gap between current cell manufacturing capabilities and the expected demand once products are approved for marketing. Current manufacturing processes are manually operated, with many open manipulations and poor understanding and control over critical process parameters. Due to these gaps, current manufacturing processes are more of an art than industrialized reproducible processes. The cell therapy industry must learn from the protein manufacturing industry and invest in process development, automation and general manufacturing science right from the beginning of product development in the pre-clinical stages. Industry must develop tools for perusing new process knowledge and understanding the process and product boundaries. On the other hand, cell therapy companies must pressure device manufacturers to develop new technologies that will allow for closed cell handling, in process CQA’s measurement and process automation for different units of operation within the manufacturing process. Industry should develop the expertise to master not only the biology side of cell therapy manufacturing, but also in process engineering, mechanical engineering, assay development and many other fields in order to become cell therapy ‘makers’. By investing the time to devise the goal of the product and then carefully analyzing the existing gaps in the process and product understanding, a successful process development campaign can be achieved – leading to the successful translation of an art to an industry.
Financial & Competing Interests Disclosure
The author is Pluristem Therapeutics Vice President of Development. No writing assistance was utilized in the production of this manuscript.
References
1. Pigeau GM, Csaszar E, Dulgar-Tulloch A. Commercial Scale Manufacturing of Allogeneic Cell Therapy. Front. Med. 2018; 5: 233. CrossRef
2. Campbell A, Brieva T, Raviv L, Rowley J, Niss K, Brandwein H, Oh S, Karnieli O. Concise review: process development considerations for cell therapy. Stem Cells Transl. Med. 2015; 4(10): 1155–63. CrossRef
3. Rowley Jon, Brieva T, Raviv L et al. Meeting lot-size challenges of manufacturing adherent cells for therapy. BioProcess Int. 2012; 10.3: 7.
4. Atouf F, Provost NM, Rosenthal FM. Standards for ancillary materials used in cell – and tissue-based therapies. BioProcess Int. 2013; 11(8).
5. Banfi A, Muraglia A, Dozin B, Mastrogiacomo M, Cancedda R, Quarto R. Proliferation kinetics and differentiation potential of ex vivo expanded human bone marrow stromal cells: implications for their use in cell therapy. Exp. Hematol. 2000; 28(6): 707e15. CrossRef
6. Tanabe S, Sato Y, Suzuki T, Suzuki K, Nagao T, Yamaguchi T. Gene expression profiling of human mesenchymal stem cells for identification of novel markers in early – and late-stage cell culture. J. Biochem. 2008; 144(3): 399e408. CrossRef
7. Zhao F, Ravindran C, Teng M. Effects of shear stress on 3‐D human mesenchymal stem cell construct development in a perfusion bioreactor system: Experiments and hydrodynamic modeling. Biotechnol. Bioeng. 2007; 96.3: 584–95. CrossRef
8. Wang H, Riha GM, Yan S et al. Shear stress induces endothelial differentiation from a murine embryonic mesenchymal progenitor cell line. Arterioscler. Thromb. Vasc. Biol. 2005; 25.9: 1817–23. CrossRef
9. Yourek G, McCormick SM, Mao JJ, Reilly GC. Shear stress induces osteogenic differentiation of human mesenchymal stem cells. Regen. Med. 2010; 5.5: 713–24. CrossRef
10. Dominici M, Le Blanc K, Mueller I et al. Minimal criteria for defining multipotent mesenchymal stromal cells. The international society for cellular therapy position statement. Cytotherapy 2006; 8(4): 315-7. CrossRef
Affiliation
Lior Raviv Vice President of Development Pluristem therapeutics Matam Park, Building 05, Haifa, 3508409, Israel Email:liorr@pluristem.com Tel.: +972-54-802-0931

This work is licensed under a Creative Commons Attribution- NonCommercial – NoDerivatives 4.0 International License.