Clinical Applications of Regulatory T cells in Adoptive Cell Therapies
Cell Gene Therapy Insights 2018; 4(1), 405-429.
10.18609/cgti.2018.042
Interest in adoptive T-cell therapies has been ignited by the recent clinical success of genetically-modified T cells in the cancer immunotherapy space. In addition to immune targeting for malignancies, this approach is now being explored for the establishment of immune tolerance with regulatory T cells (Tregs). Herein, we will summarize the basic science and clinical results emanating from trials directed at inducing durable immune regulation through administration of Tregs. We will discuss some of the current challenges facing the field in terms of maximizing cell purity, stability and expansion capacity, while also achieving feasibility and GMP production. Indeed, recent advances in methodologies for Treg isolation, expansion, and optimal source materials represent important strides toward these considerations. Finally, we will review the emerging genetic and biomaterial-based approaches on the horizon for directing Treg specificity to augment tissue-targeting and regenerative medicine.
Now over 20 years since the discovery of regulatory T cells (Tregs) within the CD4+CD25+ T cell fraction [1], investigations of adoptive Treg cellular therapies are well underway to restore or induce immunological tolerance in settings of autoimmunity, allogeneic transplantation, allergy, protein replacement therapy, graft versus host disease (GvHD), and more [2–19]. Though once thought to be a single T cell lineage, diverse Treg subsets are now identified according to an array of surface proteins, transcription factors, and mechanisms of suppression. Indeed, there may be organ-specific autoimmune disease applications where FOXP3+Helios+ thymic-derived Tregs (tTregs) are optimal for adoptive cell therapies (ACT), whereas other diseases may preferentially benefit from the bystander mechanisms employed by peripherally-induced Tregs (pTreg) (reviewed in [20]), IL-10-producing Tr1 cells (reviewed in [21]) or TGF-β secreting LAP+ Th3 cells [22]; hence, not all Tregs are equal and may need to be tailored to a given pathology.
In vitro suppression assays have been used to demonstrate the capacity for Tregs to attenuate responder cell proliferation through both antigen-specific and bystander mechanisms in a dose-dependent manner [23,24]; therefore, increasing the Treg ratio relative to pathogenic effector cells in vivo, through ACT, may bolster immunoregulation to achieve clinical benefits for immune-mediated diseases resulting from autoimmunity or allorecognition. Animal studies in various disease models have justified this rationale [25–28] and led to the initiation of human clinical trials in patients with type 1 diabetes (T1D) [2–4], Crohn’s disease [5], solid organ transplantation (i.e., liver [6] and kidney [7]), and allogeneic hematopoietic stem cell transfer (allo-HSCT) [13–18]. Herein, we review the lessons learned from these early-phase studies and the recent advances that will enable progress toward safe, efficacious, and cost-efficient Treg therapy for widespread use in the clinic.
Clinical Applications of Tregs
Tregs display wide-ranging mechanisms of action and have displayed efficacy in ameliorating a large number of inflammatory and autoimmune conditions. However, translating early proof-of-concept animal experiments into clinical practice raises a number of practical considerations, including:
- What are the specific target antigen(s)?
- Is the disease progressive or relapsing and remitting?
- Is there a potential for Tregs to reverse a disease process that is already underway?
In terms of antigen-specificity, polyclonal Tregs may be appropriate for systemic diseases such as systemic lupus erythematosus (SLE) [29], while other conditions with tissue or protein-directed pathologies might benefit from antigenic targeting via TCR or chimeric antigen receptor (CAR)-directed approaches [30,31] (discussed in greater detail below). CAR-modified T cell (CAR-T) immunotherapy has provided remarkable efficacy against various forms of cancer [32–38]. These cell-based therapies are more specifically targeted than traditional chemotherapeutic treatments, and strategies are being developed to manage the most common adverse events (e.g., cytokine release syndrome) [39]. Hence, there is clear incentive to apply these tissue-targeting principles toward ACT involving Tregs to limit potential off-target immunosuppression (Table 1).
Table 1: GMP polyclonal Treg isolation and/or expansion strategies from peripheral blood mononuclear cells or umbilical cord blood reported in the literature. | ||||||||||||||
---|---|---|---|---|---|---|---|---|---|---|---|---|---|---|
Treg Source | Isolation | Depleted Subsets | Depletion pre- or post-isolation | Activation | Stimulation (days) | Expansion time (days) | IL-2 (IU/ml) | Rapamycin | Fold-expansion | Final cell number | Purity (%) | Target Dose | Disease | Ref. |
Proof-of-concept studies | ||||||||||||||
PBMC | CliniMACS | CD8+ | Pre | Dynabeads | 0 | 10 | 25 | – | 31±16 | – | 57 | – | Autoimmunity, transplantation | [114] |
PBMC | CliniMACS | CD8+ | Pre | allogeneic PBMC | 0 | 10 | 25 | – | 28±14 | – | 54 | – | Autoimmunity, transplantation | [114] |
PBMC | CliniMACS | CD8+, CD19+ | Pre | Dynabeads | 0 | 10 | 25 | – | 34±17 | – | – | – | Autoimmunity, transplantation | [114] |
PBMC | CliniMACS | CD8+, CD19+ | Pre | allogeneic PBMC | 0 | 10 | 25 | – | 34±11 | – | – | – | Autoimmunity, transplantation | [114] |
PBMC | FACS | – | – | Dynabeads, aAPCs | 0, 9 | 14 | 300 | – | 480 | – | 92 | 0.5 × 106/kg | – | [141] |
PBMC | CliniMACS | CD8+, CD19+ | Pre | aAPCs (KT64/86) | 0, ~10, ~20, ~30, ~40 | ~45 | 300 | 109nM | 5x106 | – | 60 | – | GvHD | [125] |
PBMC | AutoMACS (CD25+) FACS | – | – | aAPCs (KT64/86) | 0, ~13, ~26, ~42, ~56 | 70 | 300 | 109nM | 31x106 | – | 63 | – | GvHD | [125] |
PBMC | CliniMACS | CD8+ | Pre | MACS ExpAct | 0, 12, 24 | 36 | 500 | 100nM | 1430–2080 | 680x106–2,680x106 | 89–91 | 4.5 × 106/kg | LT | [84] |
UCB | FACS | – | – | MACS ExpAct | 0, 9, 18 | 27 | 600 | – | 15000 | 3x1010 | >90 | 30 × 106/kg | T1D | [61] |
UCB | FACS | – | – | aAPC & Dynabeads | 0, 9 | 14 | 600 | – | 1200 | 4x109 | >90 | 30 × 106/kg | T1D | [61] |
UCB | FACS | – | – | Dynabeads | 9 | 16 | 300–600 | – | 2100 | 1.1x1010 | >90 | 30 × 106/kg | T1D | [61] |
PBMC | CliniMACS | – | – | MACS ExpAct | 0 | 21 | 500 | 100ng/ml | 22–778 | – | 90 | – | UC | [59] |
PBMC | CliniMACS | CD8+, CD19+ | Post | MACS ExpAct | 0, 14 | 21 | 1000 | 100ng/mL | 59.4–117 | 528x106 – 1,440x106 | 94 | – | Various autoimmune and inflammatory disorders | [60] |
Clinical trials of Treg adoptive cell therapy | ||||||||||||||
PBMC (Buffy Coat) | MACS/FACS | CD8+, CD19+ | – | Dynabeads | 21 | 1000 | – | – | – | >95 | 3x60x106/kg | aGvHD | [13] | |
T1D | [3] | |||||||||||||
UCB | CliniMACS | – | Dynabeads | 0 | 18±1 | 300 | – | 13–1796 | 74 × 106–12.6 × 109 | 62–97 | 0.1–3 × 106/kg 0–3 × 106/kg | aGvHD | [14] | |
dPBMC | CliniMACS | CD8+, CD19+ | Pre | – | – | – | – | – | – | – | 93 | 2–4 × 106/kg | GvHD | [15] |
PBMC | Ficoll; limiting dilution | – | – | S2 cells, expressing CD80/CD58/antiCD3, IL-2/4; OVA | 0 | 7+ | Feeder cell derived | – | – | 1 × 109 | 55 | 106–109 | CD | [5,178] |
PBMC | CliniMACS | CD8+, CD19+ | Pre | – | – | – | – | – | – | – | 81 | 2.5±1 × 106/kg | GvHD | [18] |
dPBMC | CliniMACS | CD8+ | Pre | Dynabeads, MACS ExpAct | 0, 7/8 | 7–12 | 300–1000 | 100ng/ml | 2.5–17.7 | 1.6 × 108–23 × 108 | 78–92 | 1.19–5.47 × 106/kg | cGvHD | [16] |
PBMC | FACS | – | – | Dynabeads | 0, 9 | 14 | 300 | – | 30–1367 | 0.19–13 × 109 | 76–97 | 5–2940 × 106 | T1D | [4] |
UCB | CliniMACS | – | – | aAPCs (KT64/86) | 0, 12±1 | 18±1 | 300 | – | 1352–27183 | 1.88 × 109–4.26 × 1010 | 83–95 | 3–100 × 106/kg | GvHD | [17] |
dPBMC | CliniMACS, FACS | – | – | ExpAct | 0, 7 | 13 | 1000 | – | 177 | 1.18 × 1010–2.85 × 1010 | 90 | – | Autoimmunity, transplantation | [179] |
PBMC | FACS | – | – | Dynabeads | 0, 9 | 14 | 300 | – | 300 | ≥1 × 109 | 93–97 | 319–363.8 × 106 | KT | [7] |
Treg sources were autologous PBMC, donor PBMC, autologous UCB, or donor UCB. Cell isolation was performed by CliniMACS or FACS with or without magnetic depletion of CD19+ and/or CD8+ cells pre- or post- expansion. Method and regimen for Treg activation (schedule, IL-2 dose, Rapamycin dose), subsequent fold expansion, final cell number, and purity are listed along with the target Treg dose and the proposed or tested disease application, including GvHD (cGvHD, aGvHD), LT, UC, T1D, KT, and CD. | ||||||||||||||
aGvHD: Acute graft versis host disease; aPBMC: Autologous peripheral blood mononuclear cells; aUCB: autologous umbilical cord blood; CD: Crohn’s Disease; CliniMACS: Magnetic bead-based cell separation; cGvHD: Chronic graft versus host disease; dPBMC: donor peripheral blood mononuclear cells; dUCB: Donor umbilical cord blood; FACS: Fluorescence activated cell sorting; GvHD: Graft versus host disease; KT: Kidney transplant; LT: Liver transplant; PBMC: Peripheral blood mononuclear cells; T1D: Type 1 diabetes; UC: Ulcerative colitis; UCB: Umbilical cord blood; – indicates not applicable, not performed, or not reported. |
Autoimmunity
Deficient numbers and/or functional suppression by Tregs have been reported as contributing factors in the pathogenesis of a number of autoimmune diseases (reviewed in [40]). This concept emanates from the profound systemic autoimmune syndrome observed in subjects presenting with a mutation in the Treg lineage-defining transcription factor FOXP3 resulting in immune dysregulation, polyendocrinopathy, enteropathy, and X-linked (IPEX) syndrome (reviewed in [41]). Similar autoimmune symptoms have been observed in other monogenic disorders, such as autoimmune polyendocrine syndrome type 1 (APS-1) characterized by mutations in the Autoimmune Regulator (AIRE) resulting in defects in thymic negative selection as well as impaired Treg suppression with reduced FOXP3 expression (reviewed in [42]). Genes controlling Treg development and function have also been identified as a source of heterogeneity related to immune regulatory defects in polygenic/multifactorial autoimmune diseases such as SLE, multiple sclerosis (MS), rheumatoid arthritis (RA), inflammatory bowel disease (IBD), T1D, juvenile idiopathic arthritis (JIA), and more [43–53]. Furthermore, Treg ACT has been reported to prevent or reverse autoimmunity in a variety of animal models [54–58]. Hence, human trials are planned or underway to treat a number of autoimmune and autoinflammatory conditions including ulcerative colitis (UC) [59,60] and T1D [2–4,61] (Table 1).
Early clinical trials of ex vivo expanded autologous Treg from peripheral blood of adults or children with T1D have already provided evidence of safety with few or no adverse reactions [2–4]. Importantly, infused autologous adult peripheral blood (APB) Tregs were shown to persist in the circulation of T1D patients for at least one year following transfer and were not associated with increased risk of infection [4]. Although conclusions about efficacy (i.e., the capacity to preserve endogenous β-cell function) cannot be formally demonstrated from these small cohorts, early trials have not resulted in reversal of disease. This may be due to several factors, such as disease staging (i.e., the pre-symptomatic stage of T1D [62,63] prior to clinical diagnosis may represent a more suitable therapeutic window), but may also be related to challenges such as Treg specificity, engraftment, persistence, and/or trafficking, as discussed below.
Allergy
Analogous to the findings in autoimmunity, Tregs from patients with allergic asthma are reduced in number and exhibit compromised suppressive capacity [64–66]. For this application, Treg ACT is still in the preclinical testing phase. Initial efforts were directed at adoptive transfer of allergen-specific Tregs [8] or in vivo induction of pTregs and Th3 cells via nasal delivery of soluble antigen [8,67]. Studies have since demonstrated adoptive transfer of polyclonal tTreg or polyclonal ex vivo induced Tregs to have similar efficacy in reducing allergic airway inflammation in mice [10]. More recently, Skuljec and colleagues reported that adoptive transfer of CAR-Tregs specific for carcinoembryonic antigen (CEA) expressed in lung epithelial tissues suppressed airway inflammation, mucus production, Th2 cytokine production, allergen-specific IgE production, bronchoalveolar eosinophilia, and airway hyperreactivity more effectively than polyclonal Treg therapy in a murine model of allergic asthma (Table 2) [11]. Efforts to examine models of other allergic conditions, including contact hypersensitivity and food allergy, have focused primarily on in vivo pTreg and Th3 cell induction [68–70], though studies of Treg ACT are warranted in these settings given the efficacy observed in models of allergic asthma.
Table 2: Studies of CAR-Tregs and TCR modified/enriched Tregs from mice and humans. | ||||
---|---|---|---|---|
Treg Modification | Method of Receptor Modification/Enrichment | Receptor Specificity | Disease/Model | Ref. |
TCR | Incubation w/ host allogenic splenocytes | Alloantigen | Graft-versus-host disease | [180] |
TCR | Transgenic mouse model | Ovalbumin | Colitis | [181] |
TCR | Transgenic mouse model | Beta cell antigen (BDC2.5) and pancreatic islet antigen (GAD286) | Type 1 diabetes | [25] |
TCR | Transgenic mouse model | Beta cell antigen (BDC2.5) | Type 1 diabetes | [143, 182] |
TCR | Transgenic mouse models/antigen specific beads (p31-I-Ag 7 ) | Beta cell antigen (BDC2.5), pancreatic islet antigen (GAD286), p31-I-Ag7 | Type 1 diabetes | [183] |
TCR | Incubation w/ host allogenic antigen-presenting cells | Alloantigen | Graft-versus-host disease | [184] |
TCR | Incubation w/ peptide-pulsed antigen-presenting cells | H2-Kb allopeptide | Transplantation | [185] |
TCR | Retroviral transduction | H-2Kd haplotype | Transplantation | [186] |
TCR | Donor strain–derived antigen-presenting cells | Directly and indirectly presented alloantigen | Transplantation | [187] |
TCR | Transgenic mouse model | Myelin basic protein | Multiple sclerosis | [188] |
TCR | Retroviral TCR gene transfer | Ovalbumin (OTII-TCR) | Rheumatoid Arthritis | [189] |
TCR | Lentiviral TCR gene transfer | Melanoma antigen tyrosinase | Adoptive cell therapy* | [141] |
TCR | Mixed lymphocyte reaction | HLA-DRb1 Alloantigen | Transplantation* | [190] |
TCR | Native to isolated Treg population | Ovalbumin | Crohns* | [5] |
TCR | Mixed lymphocyte reaction (CD40L-stimulated B cells) | Diverse TCR | Transplantation* | [142] |
TCR | Retroviral transduction | Factor VIII (FVIII) | Hemophilia* | [81] |
TCR | Lentiviral TCR gene transfer | Pancreatic islet antigen | Type 1 diabetes* | [30] |
TCR | Lentiviral TCR gene transfer | GAD555–567 | Type 1 diabetes* | [24] |
CAR | Transgenic mouse model | Antigen MBP89-101 | Autoimmunity (EAE) | [191, 192] |
CAR | Transgenic mouse model | 2,4,6-trinitrophenol (TNP) | Colitis | [193] |
CAR | Lentiviral transduction | Myelin oligodendrocyte glycoprotein | Multiple sclerosis | [194] |
CAR | Retroviral transduction | Carcinoembryonic antigen (CEA) | Colitis | [195] |
CAR | Lentiviral transduction | HLA-A2 | Graft Rejection* | [196] |
CAR | Retroviral transduction | HLA-A2 | Graft Rejection* | [197] |
CAR | Lentiviral transduction | HLA-A2 | Graft Rejection* | [85] |
CAR | Retroviral transduction | Factor VIII (FVIII) | Hemophilia* | [31] |
CAR | Transgenic mouse model | Carcinoembryonic antigen (CEA) | Asthma | [11] |
mAb-CAR | Transient genomic DNA plasmid transgene | Modular (Monoclonal antibody-directed) | Alloreactivity | [198] |
* Human study |
Protein replacement therapy
Inhibitory antibody formation occurs in approximately 30% of patients receiving life-saving protein replacement therapy for the blood clotting disorder hemophilia; similarly, the development of neutralizing antibodies occurs in a subset of patients during enzyme replacement therapy for monogenic lysosomal storage disorders (e.g., Pompe disease) and monogenic metabolic disorders (e.g., Fabry disease) [71–74]. Hence, Treg-mediated tolerance induction represents a critical goal to prevent/overcome inhibitor formation for successful long-term disease management (reviewed in [75,76]). In murine models of Pompe disease and hemophilia, oral delivery of plants expressing the disease-relevant antigen (i.e., acid alpha-glucosidase (GAA) or coagulation factor VIII or IX, respectively) induced Tr1, Th3, and pTreg mediated tolerance and prevented antibody inhibitor formation; however, tolerance was lost upon cessation of oral treatment [77–80]. In contrast, exogenous polyclonal Treg therapy induced durable tolerance for two months post-infusion in mice with hemophilia [12]. Recently, two coagulation factor VIII specific human Tregs applications (i.e., TCR transgenic and ASN8 CAR-Tregs) were shown to suppress cognate T and B cell responses in vitro and in a humanized mouse model (Table 2) [31,81]. As Good Manufacturing Practice (GMP) grade production of either polyclonal or antigen-specific exogenous Treg therapies becomes more streamlined, clinical investigation is clearly needed to test Tregs’ ability to prevent or suppress inhibitory antibodies in patients with Pompe disease and hemophilia. Beyond this, we anticipate that the knowledge gained from early studies in hemophilia and Pompe disease may translate effectively for a large number of rare metabolic, lysosomal, and clotting disorders for which protein replacement therapies exist [82]. In fact, these diseases may hold some potential advantages, including the capacity to control the timing of ACT therapy with protein replacement and the potential for more permissive background genetics when compared to individuals carrying autoimmune disease susceptibility alleles.
Allotransplantation & GvHD
There is a rapidly growing body of literature regarding the use of Treg ACT for applications in allotransplantation (reviewed in [83]) and GvHD. In a pilot study of ten adult liver transplant recipients, autologous polyclonal Tregs were expanded ex vivo in the presence of irradiated donor lymphocytes plus anti-CD80/86 monoclonal antibodies. Following Treg infusion, seven of the ten patients were completely weaned from immunosuppressive drugs while the remaining three continued taking low dose immunosuppression [6]. Importantly, isolation and expansion of Tregs was possible from kidney transplant recipients taking immunosuppressive drugs, and expanded polyclonal Tregs were stable, persistent, and efficacious in reducing kidney inflammation post-infusion [7]. Moreover, cryopreservation of expanded polyclonal human Tregs did not alter their phenotype or function, suggesting Tregs can be expanded, manipulated (genetically or otherwise), and banked for future use [84]. Finally, CAR-directed Tregs, specific for graft donor HLA class I, were recently shown to prevent cutaneous graft rejection in a model of xenograft transplantation, supporting human trials of these antigen-specific, “designer” Tregs in allotransplantation [85].
GvHD represents a severe and significant risk associated with allo-HSCT that confers lifelong and even terminal complications (reviewed in [86]). While first-in-man studies of Treg ACT were performed in response to GvHD [13], the first report of Treg ACT prior to allo-HSCT prevented GvHD in the absence of immunosuppressive drugs in 24/26 human leukemia patients who achieved full engraftment [15]. In a subsequent trial, co-transfusion of Tregs with conventional T cells (Tconv) and CD34+ HSCs at the time of allo-HSCT, again without immunosuppressive drugs, prevented GvHD in 85% of patients with only 0.05 cumulative incidence of relapse, suggesting that Tregs do not impede graft-versus-leukemia efficacy and provide appreciable protection against GvHD [18]. In a dose escalation trial, Tregs isolated and expanded from non-autologous umbilical cord blood (UCB) significantly reduced the incidence of both acute and chronic GvHD following double UCB transplantation compared to controls who did not receive UCB Tregs [17]. Altogether, the data support polyclonal Tregs, obtained from autologous or donor peripheral blood or UCB, as a safe and effective cellular drug in settings of GvHD.
Regeneration
Tregs, commonly thought of as mediators of immunoregulation, are now being studied for their capacity to induce tissue regeneration and remodeling. Treg therapy may enhance hepatic recovery in fulminant or chronic hepatitis, the latter of which eventually results in liver cirrhosis and liver cancer (recently reviewed in [87]). Indeed, polyclonal Treg ACT prevented liver injury and enhanced hepatic regeneration in mouse models of fulminant hepatitis [88–90]. Endogenous memory tTregs become highly enriched in the liver of human patients with chronic Hepatitis B infection during phases of inflammatory relapse, likely signifying an attempt to control hepatic injury [91]. Hence, it seems plausible that adoptive Treg therapy combined with antiviral medication might improve clinical outcomes and reduce liver damage in patients with viral hepatitis; however, there is an ongoing debate as to whether Tregs might potentiate chronic infection by suppressing antiviral immunity, necessitating further investigation (reviewed in [92,93]).
A specific subset of muscle-resident Tregs expressing amphiregulin and IL-10 has been shown to support muscle regeneration and repair following acute injury [94]. Moreover, Treg depletion exacerbated muscle degeneration in the mdx mouse model of muscular dystrophy while Treg potentiating treatment with low-dose IL-2 reduced muscle inflammation [95]. Muscle biopsies from human muscular dystrophy patients exhibit increased Treg frequencies versus healthy controls, similar to mdx versus control mice, suggesting endogenous repair mechanisms are insufficient to ameliorate the disease [95]. Hence, there is potential for ACT with Tregs modified to overexpress amphiregulin or other regenerative factors to support tissue repair in settings of injury or chronic degenerative disease such as muscular dystrophy.
Practical Considerations
Treg-based therapies hold great promise for inducing tolerance without many of the known toxicities observed with global immunosuppessive drugs (reviewed in [96–99]). In order to make Treg therapies feasible for widespread clinical use, it is essential to optimize the pipeline from isolation and expansion to infusion.
Treg purity of the final cell product will be a major factor determining therapeutic efficacy. Since most Treg therapy production strategies are likely to include expansions, elimination of non-Treg contaminants during the isolation step (Figure 1) is the most efficient way to achieve the purest product. In addition, specific Treg lineage and source (i.e., UCB Tregs or naïve peripheral blood Tregs, discussed below) may be considered when designing an enrichment and purification approach. Therefore, lineage and source selection, as well as isolation and enrichment strategies must be optimized to minimize the incidence of unintended immune activation and inflammation induced by the Treg ACT. Herein, we will discuss isolation strategies capable of generating a high yield and purity Treg product.
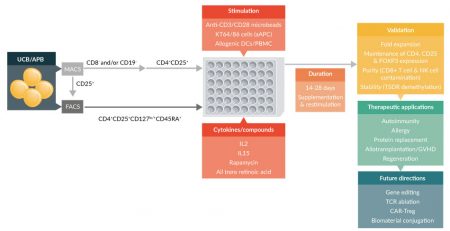
Figure 1: Ex vivo human Treg expansion protocols reported in the literature. Isolation of Tregs from UCB or APB is accomplished by either MACS or FACS. FACS is often used to sort out an enriched population of CD4+CD25+CD127lo/-CD45RA+ cells directly from the starting sample, but MACS can be employed prior to sorting to remove contaminating CD8+ and CD19+ cell types [84,114,125]. Likewise, MACS can be utilized to pre-enrich for CD25+ cells prior to FACS to obtain a highly enriched population of CD4+CD25+CD127lo/-CD45RA+ cells. Regardless of the isolation protocol, these highly enriched cells are then cultured in the presence of cytokines to support Treg proliferation and inhibitory compounds such as rapamycin to maintain Treg purity. Expansion of the Treg population is dependent on the introduction of stimulatory agents in the form of either anti-CD3/CD28 monoclonal antibody coated beads, allogenic DCs, or artificial APCs. Expansion of Treg populations requires up to 28 days of culture with multiple re-stimulations and frequent cytokine supplementation of the growth media. Expanded cells are validated for fold expansion, stable expression of Treg markers, purity, and stability before being used in therapeutic applications or experimental studies designed to enhance Treg specificity and potency.
Identification of human Treg
FOXP3 serves as a master regulator of Treg lineage commitment and function. In human T cells, however, it alone does not define a Treg cell given that Tconv also transiently express intermediate levels of FOXP3 following activation [100]. FOXP3 expression in combination with another transcriptional regulator, Helios, denotes a thymic-derived tTreg population noted for phenotypic stability, suppressive potency, and ability to bestow these qualities unto daughter cell generations [101,102]. Within tTreg, the high degree of demethylation of the Treg-specific demethylated region (TSDR), found within the conserved non-coding DNA sequence 2 (CNS2) enhancer in the FOXP3 locus confers lineage stability [101–105]. Additionally, tTregs express homing receptors, including chemokine receptors and adhesion molecules that enable trafficking both systemically and to disease-relevant tissues [106–110]. As compared to pTreg populations, tTregs exhibit a higher degree of lineage stability upon in vivo transfer [101,104]. Hence, tTregs are commonly targeted for ACT, though head-to-head comparisons of Treg subsets are ongoing to determine which subset may be optimal in certain disease settings (reviewed in [111]).
With the identification of FOXP3+Helios+ Tregs, numerous advances in our understanding of immune regulation have been achieved. Unfortunately, labeling intracellular proteins (including these transcription factors) renders cells inviable; therefore, Treg isolation must rely on surface markers. In 2007, human Tregs were found to express low surface levels of the alpha chain of the IL-7 receptor, CD127, compared to Tconv [112], thereby enabling identification and isolation of live Treg based on the CD3+CD4+CD25+CD127-/lo phenotype [61]. Importantly, cells isolated by this phenotype are highly enriched for FOXP3+Helios+ co-expression [113]. This observation represented a key step enabling future Treg cellular therapies in humans, given the ostensible importance of purity prior to reinfusion.
Human Treg isolation
Currently two methods exist for live Treg isolation: magnetic bead-based isolation or fluorescence-activated cell sorting (FACS). The former involves the closed-system isolation of CD4+CD25+ T cells and was widely used for early trials, given prior experience with CD34+ HSC sorting and ACT. Strategies can be implemented to increase Treg purity from magnetic isolation such as: 1) depletion of CD8+ and CD19+ subsets before Treg isolation [15,16,18] or from the final post-expansion product [60]; 2) depletion of CD127+ population [114]; 3) perform multiple rounds of CD25+-selection; or 4) limit the amount of anti-CD25 antibody loaded on the bead [115]. Unfortunately, these strategies dramatically reduce the overall yield and fail to eliminate activated and memory CD4+CD25+ populations from the final product. Generally, this can be overcome by addition of rapamycin and/or all trans retinoic acid (ATRA) to the Treg expansion media, which inhibit Tconv proliferation and promote conversion of naïve T cells to induced Tregs, respectively [116].
FACS allows for precise gating based on marker expression level in contrast to microbead bulk enrichments. Importantly, emerging GMP-compliant, closed-loop sorting systems for FACS are expected to enable optimized isolation of CD4+CD25+CD127-/lo Tregs. Soon to follow will likely be GMP-compatible reagents enabling the identification of precise cell subsets, potentially based on yet-to-be-defined surface proteins or technologies relying on transcriptional markers of phenotype [117]. For example, it is well recognized that CD4+CD25+CD127-/loCD45RA+ naïve Tregs exhibit greater stability during ex vivo expansion, while CD4+CD25+CD127-/loCD45RA– memory Tregs are more likely to downregulate FOXP3 and upregulate their expression of proinflammatory cytokines [118–120]. We recently demonstrated that the co-inhibitory receptor T-cell immunoglobulin and immunoreceptor tyrosine-based inhibitory motif domain (TIGIT) and Helios were highly co-expressed by tTregs [121]. Conversely, CD226, which directly competes with TIGIT to bind CD155, was most highly expressed by Tconv and FOXP3+Helios– pTregs. Of note, CD226 depleted Tregs exhibited comparable expansion kinetics and enhanced suppressive capacity compared to non-depleted cells. Eventually, protocols will narrow until the most functionally appropriate Treg, perhaps tailored to the disease/condition, can be delivered in the safest manner.
Expansion approaches
The number of peripheral blood mononuclear cells (PBMCs) that can be safely obtained from patients via peripheral blood draw or leukapheresis is limited according to body weight. Hence, obtaining clinically relevant numbers of Tregs sufficient to induce immunoregulation likely requires ex vivo expansion. Optimal conditions for Treg expansion involve multiple (i.e., 1-3) rounds of re-stimulation over the course of a 14-28 day culture in the presence of exogenous IL-2 [122–126]. Culture durations beyond this time frame are contraindicated because subsequent rounds of activation can compromise Treg suppressive function and stability [119]. Various stimulation approaches exist (i.e., antigen presenting cells [APCs] loaded with antigen, artificial APCs [aAPCs], allo-APCs, beads), each offering unique merits (Table 1, Figure 1).
The earliest aAPCs (K562 cells) are well described in the literature [127–129]. Briefly, K562 cells have been genetically modified to achieve long-term and stable expression of human CD32 and CD64 (KT32/64), which together bind the Fc region of anti-CD3 (OKT3) antibodies; hence, K562 aAPCs are capable of inducing robust Treg proliferation [128]. Moreover, Tregs stimulated through the CD28 pathway (by KT32/86 cells that constitutively express CD86) in addition to the CD3 pathway and in the presence of rapamycin showed 1000-fold expansion in three weeks with consistent suppression of xenogeneic GvHD in immunodeficient mice [130]. Cell-based aAPC expansion approaches provide a standardized platform for consistent Treg expansion and thus, are attractive for therapeutic applications.
Importantly, anti-CD3 and anti-CD28 coated microbeads offer an off-the-shelf GMP-compliant alternative to APC-based expansion. Just two rounds of activation (on day 0 and day 9) and 300U/ml IL-2 replenishment every two days routinely achieved >1000 fold Treg expansions [131,132]. We have also found that different GMP-grade products provide varying activation thresholds for TCR activation and co-stimulation impacting both the expansion efficiency and stability of the final Treg product [132].
Treg expansion capacity and lineage stability
The need to generate a sufficiently large, pure Treg population represents a significant challenge in Treg ACT. The optimal Treg dose is unknown and likely differs for each disease application. However, in mice that received a solid organ transplant, tolerance was achieved when approximately 30% of the organ-infiltrating CD4+ T cells were Tregs [133]. Supposing this value might translate to Treg cell therapy in settings of autoimmunity, Tang et al. calculated a theoretical target dose of 53×109 Treg to achieve a systemic Treg frequency of 30% among total CD4+ T cells in human patients [134]. Such a high dose is likely unfeasible due to practical/technical limitations and far exceeds the highest doses tested to date (100 × 106 Treg/kg [17]) but may support cryopreservation of Treg cells for subsequent repeat dosing. Additionally, efforts to optimize Treg ACT by selecting optimal cell sources and/or modulating their specificity, trafficking, or function, prior to reinfusion are ongoing in order to circumvent this potential limitation.
Efforts to expand either peripheral blood [4,7] or UCB-derived Tregs [61] have yielded cellular products that far surpass clinical release criteria for viability and/or CD8+ T cell contamination [4,7]. However, we recently reported that post-expansion Treg stability (i.e., FOXP3 and Helios co-expression as well as TSDR demethylation), purity (calculated from CD8+ T cell contamination), and Treg naivety (assessed by CD45RA expression) were significantly greater when Tregs were derived from UCB versus adult peripheral blood (APB) [61]. UCB Tregs also exhibited greater proliferative capacity compared to APB Tregs [61]. Moreover, expansion kinetics and post-expansion assessments (i.e., purity, stability, naivety) were comparable for Tregs isolated from fresh or cryopreserved UCB [61]. Hence, UCB may represent an optimal Treg source for ACT, eliminating the need for leukapheresis procedures, with cryopreserved UCB offering the potential for autologous Treg treatment.
Treg Specificity
Tregs are classically considered to modulate Tconv responses through both antigen-dependent and bystander suppression mechanisms [24], but they also exert their regulatory effects on the innate immune cells [135,136]. As a result, polyclonal Tregs may be particularly suitable for treatment of autoimmune or autoinflammatory conditions where the target antigen remains unknown or where a variety of autoantigens/tissues are targeted [137,138], but they might also offer a valuable treatment option for organ-specific diseases without the need for extensive ex vivo manipulation [4,7]. In contrast, antigen-specific Tregs, generated through TCR gene transfer, introduction of a CAR or selective expansion (Table 2), may traffic more efficiently to the site of cognate antigen expression and tissue draining lymph nodes [139–141], making it possible to achieve an efficient dose of Tregs in the target tissue/organ with a lower systemic Treg frequency. In a humanized mouse model of allotransplantation, selectively expanded alloantigen-reactive autologous Tregs were more effective at preventing allograft rejection compared to polyclonally expanded autologous Tregs [142]. Similarly, compared to polyclonal Tregs, 10-fold fewer β-cell antigen-specific Tregs were able to reverse autoimmune diabetes in NOD mice [143]. However, it is important to consider that in human T1D various epitopes are targeted, including native β-cell antigens (i.e., insulin, GAD, IA-2, ZnT8) as well as hybrid insulin peptides (HIPs) and defective ribosomal insulin gene products (DRiPs) [144–147], so polyclonal or oligoclonal Treg preparations may provide greater autoantigen coverage. Moreover, we recently demonstrated that two TCRs reactive against the same peptide epitope with different avidities exhibited significantly different capacity to suppress Tconv [24].
Additional considerations, such as location of antigen expression/mode of antigen presentation, must guide receptor selection for antigen-specific Treg ACT. TCR redirected Tregs offer the benefit of recognizing intracellular antigen presented in the context of HLA, but the requirement for HLA compatibility requires use of autologous cells. In contrast, CAR-Tregs, which express the antigen binding region of an immunoglobulin (Ig) fused to intracellular T cell signaling domains [37,38], do not engage HLA but rather, recognize extracellular antigen. Extensive studies of CAR-T cells in the cancer field have set the stage for CAR and TCR-redirected Tregs for ACT (summarized in Table 2). Ultimately, clinical studies are needed to identify the most appropriate Treg application (i.e., polyclonal or antigen-specific and TCRs or CARs) for use in various disease states.
Engraftment & trafficking
While the efficacious Treg dose has yet to be determined for the treatment of autoimmunity, a dose escalation study in adults with T1D demonstrated an exceptional safety profile even with high doses (up to 2.6×109 cells) of ex vivo expanded autologous peripheral blood Treg. Importantly, Bluestone et al. reported that Tregs labeled with deuterated glucose were still detectable from circulation 12 months following transfer [4]. This suggests Tregs may display a similar long-term engraftment potential observed in cancer immunotherapy settings [148–151]. Additionally, IL-2 adjunct therapy may enhance Treg engraftment, similar to the effect of Ibrutinib with CAR-T treatment of chronic lymphocytic leukemia [152].
Ex vivo expanded human Tregs were shown to downregulate their expression of CCR5 [153], which directs T cell homing to sites of inflammation [154,155]. In contrast, human T cells exposed to the vitamin D analogue TX527 exhibited upregulated expression of the inflammatory homing receptors, CCR5, CXCR6, and CXCR3 [156]. CCR4+ human Treg migration into ovarian tumors was dependent upon CCL22 [157]. Furthermore, in a murine model of IBD, adoptive transfer of CCR4 competent Tregs prevented colitis, but CCR4 deficient Tregs were unable to traffic to the mesenteric lymph nodes resulting in disease [158]. Modification of chemokine receptor(s) and integrin expression could further guide Treg trafficking post-infusion. These approaches theoretically offer the additional benefit of reducing the likelihood of off-target suppression, which might impair protective immunity against an infection or cancer.
Regulatory requirements
Regulatory agencies, such as the U.S. Food and Drug Administration (FDA) and the European Medicines Agency [159], provide several guidelines about the cell therapy manufacturing process to ensure that each batch meets stringent quality standards concerned with cell collection, manufacturing area, the instruments and reagents used, and the release criteria of the final product prior to application. In addition to passing sterility screenings conducted throughout isolation and expansion, the final Treg product must satisfy purity criteria (e.g., composition of 60% FOXP3+ Treg; or >96.7% cell viability, 99.6% CD4+, 98.9% CD25+, 0.0% CD127+, 0% CD8+, 0.0% CD19+, and 0.0% CD56/16+) [4,60] and potentially, confirmation of functional suppression in vitro [60] prior to infusion. Thought must also be given to the timing of Treg infusion, particularly in children, with relation to vaccination schedules.
For research use, FACS occurs in an open system; therefore, the clinical application requires a modified, closed instrument, in Grade A “clean rooms”, equipped with high efficiency particulate air (HEPA) filters to limit air particulates and avoid microbial contamination. In contrast, cell isolation using CliniMACS (Miltenyi) is performed in a closed system with disposable tubing limiting the potential for contamination inherent in droplet based FACS approaches. As a result, magnetic bead-based isolation currently offers better affordability and a shorter cell sorting process but is limited to basic bimodal sorts. However, as mentioned above, emergent closed-loop FACS technologies are highly anticipated. Indeed, this interface will enable more widespread application of cellular therapies offering more precise and affordable GMP isolation of CD4+CD25+CD127-/lo Tregs for a highly pure cell product.
Future Directions for Treg Cellular Therapy
Moving forward, advances in isolation, expansion, and cellular engineering technologies are expected to enable precise engineering of optimal Treg products for various therapeutic applications (reviewed in [160]). Gene editing techniques, such as endogenous TCR ablation, are expected to reduce potential heterologous chain pairing. Moreover, allogeneic cell sources for TCR-redirected Treg or CAR-Treg therapies could eliminate the need for invasive leukapheresis procedures. Given the inherently different argument for equipoise in chronic conditions such as autoimmunity and allergy versus terminal cancer, efforts are underway to introduce additional safeguards, including suicide genes [37,161,162], to enable depletion of the cells post-infusion in the event of off target suppression or an unforeseen adverse reaction [161,162].
Gene editing could theoretically be used to optimize “designer Tregs” via knockdown of molecules known to inhibit suppressive function, introduction of regulatory elements (e.g., TIGIT [121,163]), expression of growth factors (e.g., amphiregulin [94,95]), and/or site-specific mutagenesis of single nucleotide polymorphism (SNPs) associated with disease risk/pathogenesis specific to the intended application. For example, Tregs from donors carrying a SNP risk variant in protein tyrosine phosphatase, non-receptor type (PTPN22), which confers risk in several autoimmune disorders and outside of HLA and insulin, has the highest odds ratio for T1D (reviewed in [164]), can be gene-edited to the protective variant prior to ex vivo expansion. Ectopic overexpression of FOXP3 in autologous Tconv could transform our ability to treat IPEX syndrome (reviewed in [165]). Additionally, we envision that it may eventually be possible to treat both allograft rejection and autoimmunity in pancreas or islet transplant recipients with T1D via ACT of CAR-Tregs against donor HLA plus polyclonal or TCR redirected Tregs specific for β-cell autoantigens, albeit likely in combination with additional immunomodulatory agents.
Our lab and others are currently pursuing biomaterials applications to optimize Treg survival and function in vivo following infusion. Poly(d-lactide-co-glycolide) (PLGA) is degraded in the body via bulk erosion and hydrolysis into glycolic acid and lactic acid, conferring no harmful side effects [166]; consequently, PLGA is used in numerous devices/therapeutics approved by the FDA, such as biodegradable surgical sutures and drug delivery products, which makes it extremely attractive for development of products quickly translatable to clinical use. PLGA can be used to produce micro- or nanoparticles (NPs), which have been widely investigated for delivering immunotherapeutics [167,168]. By altering the lactide/glycolide ratio, PLGA NPs can be designed to provide tunable sustained release of encapsulated immunomodulatory molecules [169]. Moreover, NPs can be engineered to simultaneously deliver therapeutic factors and adjuvant drugs, each with tailored release kinetics while maintaining the tissue-targeting capacity of a CAR or TCR. Indeed, controlled release of immunomodulatory agents by PLGA particulate systems are being explored as immunotherapeutic tools for treatment of cancer, autoimmunity and transplant-related complications [170–172]. An attractive strategy is to focus drug and growth factor action on adoptively-transferred, therapeutic Tregs through the conjugation of agent-loaded NPs directly onto the cell surface via the incorporation of reactive groups into the PLGA formulation [173]. This flexible approach allows for concurrent in vivo delivery of therapeutic agents in both an autocrine and paracrine manner. As result, it may be possible to simultaneously conjugate Tregs to PLGA NPs loaded with Treg growth factors (e.g., IL-2) and to PLGA NPs loaded with therapeutic agents designed to impact the extracellular milieu upon Treg migration to sites of inflammation.
Clearly, a vast number of possible targets for cellular manipulation exist, supporting a synthetic biology approach to therapeutic T cell engineering [174]. By establishing a standardized series of ex vivo modifications for each target tissue/disease application, scientists and clinicians will be able to ensure infusion of a consistent therapeutic product, essential for both safety and efficacy. The feasibility of using Tregs as a future ACT to restrain inflammation is supported by numerous studies utilizing either allogeneic or autologous effector T cells to augment immunity in cancer patients (reviewed in [175–177]). The therapeutic potential of these cells has been further augmented by genetic engineering, conferring upon T cells the ability to target a specific tumor-associated antigen while simultaneously increasing their lifespan and reducing potential toxicity [32–38]. As a result, ACT-based immunotherapies have been extraordinarily successful in treating cancer and infectious diseases, and the lessons learned from those efforts will afford an advantage toward the design and implementation of novel Treg therapies.
Author Contributions
GPM, ALP, JC and TMB wrote the manuscript. GPM created the figure. DJP, WIY, HRS and AGE reviewed/edited the manuscript and TMB conceived the paper.
Conflict of Interest
GPM is the senior scientist and TMB is an equity owner and chief operational officer (COO) at OneVax, LLC. OneVax is a preclinical research company investigating biomaterial-based Treg cellular therapies for the treatment of autoimmune diseases. OneVax did not grant monetary compensation for the perspectives conveyed in this manuscript, nor do they benefit from the manuscript’s publication. The remaining authors declare that no financial conflicts of interest exist.
Funding Statement
These efforts were supported by grants from the National Institutes of Health R01 DK106191 (TMB), National Institutes of Health R21 AI133067-01 (GPM), National Institutes of Health R43 AI131850-01 (GPM), JDRF Career Development Award 2-2012-280 (TMB), and JDRF Postdoctoral Fellowship Award 2-PDF-2016-207-A-N (DJP).
Guarantor Statement
Todd M. Brusko is the guarantor of this work and as such, assumes full responsibility for the ethical acquisition and reporting of information.
References
1. Sakaguchi S, Sakaguchi N, Asano M, Itoh M, Toda M. Immunologic self-tolerance maintained by activated T cells expressing IL-2 receptor alpha-chains (CD25). Breakdown of a single mechanism of self-tolerance causes various autoimmune diseases. J. Immunol. 1995; 155: 1151–1164.
2. Marek-Trzonkowska N, Mysliwiec M, Dobyszuk A et al. Administration of CD4+CD25highCD127- regulatory T cells preserves β-cell function in type 1 diabetes in children. Diabetes Care 2012; 35: 1817–1820.
CrossRef
3. Marek-Trzonkowska N, Mysliwec M, Siebert J, Trzonkowski P. Clinical application of regulatory T cells in type 1 diabetes. Pediatr. Diabetes 2013; 14: 322–332.
CrossRef
4. Bluestone JA, Buckner JH, Fitch M et al. Type 1 diabetes immunotherapy using polyclonal regulatory T cells. Sci. Transl. Med. 2015; 7: 315ra189.
CrossRef
5. Desreumaux P, Foussat A, Allez M, Beaugerie L et al. Safety and efficacy of antigen-specific regulatory T-cell therapy for patients with refractory Crohn’s disease. Gastroenterology 2012; 143: 1207–1217. e1201–1202.
6. Todo S, Yamashita K, Goto R et al. A pilot study of operational tolerance with a regulatory T-cell-based cell therapy in living donor liver transplantation. Hepatology 2016; 64: 632–643.
CrossRef
7. Chandran S, Tang Q, Sarwal M et al. Polyclonal Regulatory T Cell Therapy for Control of Inflammation in Kidney Transplants. Am. J. Transplant. 2017; 17: 2945–2954.
CrossRef
8. Kearley J, Barker JE, Robinson DS, Lloyd CM. Resolution of airway inflammation and hyperreactivity after In vivo transfer of CD4+CD25+ regulatory T cells is interleukin 10 dependent. J. Exp. Med. 2005; 202: 1539–1547.
CrossRef
9. Kearley J, Robinson DS, Lloyd CM. CD4+CD25+ regulatory T cells reverse established allergic airway inflammation and prevent airway remodeling. J. Allergy Clin. Immunol. 2008; 122: 617–624.e616
CrossRef
10. Xu W, Lan Q, Chen M et al. Adoptive transfer of induced-Treg cells effectively attenuates murine airway allergic inflammation. PLoS One 2012; 7: e40314.
CrossRef
11. Skuljec J, Chmielewski M, Happle C et al. Chimeric Antigen Receptor-Redirected Regulatory T Cells Suppress Experimental Allergic Airway Inflammation, a Model of Asthma. Front. Immunol. 2017; 8: 1125.
CrossRef
12. Sarkar D, Biswas M, Liao G et al. Ex vivo Expanded Autologous Polyclonal Regulatory T Cells Suppress Inhibitor Formation in Hemophilia. Mol. Ther. Methods Clin. Dev. 2014; 1.
CrossRef
13. Trzonkowski P, Bieniaszewska M, Juscinska J et al. First-in-man clinical results of the treatment of patients with graft versus host disease with human ex vivo expanded CD4+CD25+CD127- T regulatory cells. Clin. Immunol. 2009; 133: 22–26.
CrossRef
14. Brunstein CG, Miller JS, Cao Q et al. Infusion of ex vivo expanded T regulatory cells in adults transplanted with umbilical cord blood: safety profile and detection kinetics. Blood 2011; 117: 1061–1070.
CrossRef
15. Di Ianni M, Falzetti F, Carotti A et al. Tregs prevent GVHD and promote immune reconstitution in HLA-haploidentical transplantation. Blood 2011; 117: 3921–3928.
CrossRef
16. Theil A, Tuve S, Oelschlägel U et al. Adoptive transfer of allogeneic regulatory T cells into patients with chronic graft-versus-host disease. Cytotherapy 2015; 17: 473–486.
CrossRef
17. Brunstein CG, Miller JS, McKenna DH et al. Umbilical cord blood-derived T regulatory cells to prevent GVHD: kinetics, toxicity profile, and clinical effect. Blood 2016; 127: 1044–1051.
CrossRef
18. Martelli MF, Di Ianni M, Ruggeri L et al. HLA-haploidentical transplantation with regulatory and conventional T-cell adoptive immunotherapy prevents acute leukemia relapse. Blood 2014; 124: 638–644.
CrossRef
19. Marek-Trzonkowska N, Mysliwiec M, Dobyszuk A, et al. Therapy of type 1 diabetes with CD4(+)CD25(high)CD127-regulatory T cells prolongs survival of pancreatic islets – results of one year follow-up. Clin. Immunol. 2014; 153: 23–30.
CrossRef
20. Yadav M, Stephan S, Bluestone JA. Peripherally induced tregs – role in immune homeostasis and autoimmunity. Front. Immunol. 2013; 4: 232.
CrossRef
21. Zeng H, Zhang R, Jin B, Chen L. Type 1 regulatory T cells: a new mechanism of peripheral immune tolerance. Cell Mol. Immunol. 2015; 12: 566–571.
CrossRef
22. Gandhi R, Farez MF, Wang Y et al. Cutting edge: human latency-associated peptide+ T cells: a novel regulatory T cell subset. J. Immunol. 2010; 184: 4620–4624.
CrossRef
23. Tang Q, Bluestone JA. The Foxp3+ regulatory T cell: a jack of all trades, master of regulation. Nat Immunol 2008; 9: 239–244.
CrossRef
24. Yeh WI, Seay HR, Newby B et al. Avidity and Bystander Suppressive Capacity of Human Regulatory T Cells Expressing De Novo Autoreactive T-Cell Receptors in Type 1 Diabetes. Front. Immunol. 2017; 8: 1313.
CrossRef
25. Tang Q, Henriksen KJ, Bi M et al. In vitro-expanded antigen-specific regulatory T cells suppress autoimmune diabetes. J. Exp. Med. 2004; 199: 1455–1465.
CrossRef
26. Parmar S, Liu X, Tung SS et al. Third-party umbilical cord blood-derived regulatory T cells prevent xenogenic graft-versus-host disease. Cytotherapy 2014; 16: 90–100.
CrossRef
27. McDonald-Hyman C, Flynn R, Panoskaltsis-Mortari A et al. Therapeutic regulatory T-cell adoptive transfer ameliorates established murine chronic GVHD in a CXCR5-dependent manner. Blood 2016; 128: 1013–1017.
CrossRef
28. Mottet C, Uhlig HH, Powrie F. Cutting edge: cure of colitis by CD4+CD25+ regulatory T cells. J. Immunol. 2003; 170: 3939–3943.
CrossRef
29. Scalapino KJ, Tang Q, Bluestone JA, Bonyhadi ML, Daikh DI. Suppression of disease in New Zealand Black/New Zealand White lupus-prone mice by adoptive transfer of ex vivo expanded regulatory T cells. J. Immunol. 2006; 177: 1451–1459.
CrossRef
30. Hull CM, Nickolay LE, Estorninho M et al. Generation of human islet-specific regulatory T cells by TCR gene transfer. J. Autoimmun 2017; 79: 63–73.
CrossRef
31. Yoon J, Schmidt A, Zhang AH, Konigs C, Kim YC, Scott DW. FVIII-specific human chimeric antigen receptor T-regulatory cells suppress T- and B-cell responses to FVIII. Blood 2017; 129: 238–245.
CrossRef
32. Zhao Y, Moon E, Carpenito C et al. Multiple injections of electroporated autologous T cells expressing a chimeric antigen receptor mediate regression of human disseminated tumor. Cancer Res. 2010; 70: 9053–9061.
CrossRef
33. Kochenderfer JN, Wilson WH, Janik JE et al. Eradication of B-lineage cells and regression of lymphoma in a patient treated with autologous T cells genetically engineered to recognize CD19. Blood 2010; 116: 4099–4102.
CrossRef
34. Gill S, June CH. Going viral: chimeric antigen receptor T-cell therapy for hematological malignancies. Immunol. Rev. 2015; 263: 68–89.
CrossRef
35. Lee DW, Kochenderfer JN, Stetler-Stevenson M et al. T cells expressing CD19 chimeric antigen receptors for acute lymphoblastic leukaemia in children and young adults: a phase 1 dose-escalation trial. Lancet 2015; 385: 517–528.
CrossRef
36. DeSelm CJ, Tano ZE, Varghese AM, Adusumilli PS. CAR T-cell therapy for pancreatic cancer. J. Surg. Oncol. 2017; 116(1): 63–74.
CrossRef
37. Jaspers JE, Brentjens RJ. Development of CAR T cells designed to improve antitumor efficacy and safety. Pharmacol. Ther. 2017; 178: 83–91.
CrossRef
38. Scarfo I, Maus MV. Current approaches to increase CAR T cell potency in solid tumors: targeting the tumor microenvironment. J. Immunother. Cancer 2017; 5: 28.
CrossRef
39. Brudno JN, Kochenderfer JN. Toxicities of chimeric antigen receptor T cells: recognition and management. Blood 2016; 127: 3321–3330.
CrossRef
40. Tao JH, Cheng M, Tang JP, Liu Q, Pan F, Li XP. Foxp3, Regulatory T Cell, and Autoimmune Diseases. Inflammation 2017; 40: 328–339.
CrossRef
41. Bacchetta R, Barzaghi F, Roncarolo MG. From IPEX syndrome to FOXP3 mutation: a lesson on immune dysregulation. Ann. NY Acad. Sci. 2016; 1417(1): 5-22
CrossRef
42. Abramson J, Husebye ES. Autoimmune regulator and self-tolerance – molecular and clinical aspects. Immunol. Rev. 2016; 271: 127–140.
CrossRef
43. Liu MF, Wang CR, Fung LL, Wu CR. Decreased CD4+CD25+ T cells in peripheral blood of patients with systemic lupus erythematosus. Scand. J. Immunol. 2004; 59: 198–202.
CrossRef
44. Borsellino G, Kleinewietfeld M, Di Mitri D et al. Expression of ectonucleotidase CD39 by Foxp3+ Treg cells: hydrolysis of extracellular ATP and immune suppression. Blood 2007; 110: 1225–1232.
CrossRef
45. Lawson CA, Brown AK, Bejarano V et al. Early rheumatoid arthritis is associated with a deficit in the CD4+CD25high regulatory T cell population in peripheral blood. Rheumatology (Oxford) 2006; 45: 1210–1217.
CrossRef
46. Li Z, Arijs I, De Hertogh G et al. Reciprocal changes of Foxp3 expression in blood and intestinal mucosa in IBD patients responding to infliximab. Inflamm. Bowel Dis. 2010; 16: 1299–1310.
CrossRef
47. Marwaha AK, Panagiotopoulos C, Biggs CM et al. Pre-diagnostic genotyping identifies T1D subjects with impaired Treg IL-2 signaling and an elevated proportion of FOXP3+IL-17+ cells. Genes Immun. 2017; 18: 15–21.
CrossRef
48. Lindley S, Dayan CM, Bishop A et al. Defective suppressor function in CD4(+)CD25(+) T-cells from patients with type 1 diabetes. Diabetes 2005; 54: 92–99.
CrossRef
49. Maggi L, Cosmi L, Simonini G, Annunziato F, Cimaz R. T cell subpopulations in juvenile idiopathic arthritis and their modifications after biotherapies. Autoimmun. Rev. 2016; 15: 1141–1144.
CrossRef
50. Onengut-Gumuscu S, Chen WM, Burren O et al. Type 1 Diabetes Genetics C, Todd JA, Wallace C, Concannon P, Rich SS. Fine mapping of type 1 diabetes susceptibility loci and evidence for colocalization of causal variants with lymphoid gene enhancers. Nat. Genet. 2015; 47: 381–386.
CrossRef
51. Worthington J, Thomson W. Investigation of type 1 diabetes and coeliac disease susceptibility loci for association with juvenile idiopathic arthritis. Ann. Rheum. Dis. 2010; 69: 2169–2172.
CrossRef
52. Dendrou CA, Plagnol V, Fung E et al. Cell-specific protein phenotypes for the autoimmune locus IL2RA using a genotype-selectable human bioresource. Nat. Genet. 2009; 41: 1011–1015.
CrossRef
53. Eleftherohorinou H, Wright V, Hoggart C et al. Pathway analysis of GWAS provides new insights into genetic susceptibility to 3 inflammatory diseases. PLoS One 2009; 4: e8068.
CrossRef
54. Delville M, Six E, Bellier F. Generation of Functional Regulatory T Cells By FOXP3 Gene Transfer into CD4 T Cells from Scurfy Mice and IPEX Patients. Blood 2016; 128: 2526.
55. Mahne AE, Klementowicz JE, Chou A, Nguyen V, Tang Q. Therapeutic regulatory T cells subvert effector T cell function in inflamed islets to halt autoimmune diabetes. J. Immunol. 2015; 194: 3147–3155.
CrossRef
56. Tonkin DR, He J, Barbour G, Haskins K. Regulatory T cells prevent transfer of type 1 diabetes in NOD mice only when their antigen is present In vivo. J. Immunol. 2008; 181: 4516–4522.
CrossRef
57. Souroujon MC, Aricha R, Feferman T, Mizrachi K, Reuveni D, Fuchs S. Regulatory T cell-based immunotherapies in experimental autoimmune myasthenia gravis. Ann. NY Acad. Sci. 2012; 1274: 120–126.
CrossRef
58. Huter EN, Stummvoll GH, DiPaolo RJ, Glass DD, Shevach EM. Cutting edge: antigen-specific TGF beta-induced regulatory T cells suppress Th17-mediated autoimmune disease. J. Immunol. 2008; 181: 8209–8213.
CrossRef
59. Voskens CJ, Fischer A, Roessner S et al. Characterization and Expansion of Autologous GMP-ready Regulatory T Cells for TREG-based Cell Therapy in Patients with Ulcerative Colitis. Inflamm. Bowel Dis. 2017; 23: 1348–1359.
CrossRef
60. Wiesinger M, Stoica D, Roessner S et al. Good Manufacturing Practice-Compliant Production and Lot-Release of Ex vivo Expanded Regulatory T Cells As Basis for Treatment of Patients with Autoimmune and Inflammatory Disorders. Front. Immunol.
CrossRef
61. Seay HR, Putnam AL, Cserny J et al. Expansion of Human Tregs from Cryopreserved Umbilical Cord Blood for GMP-Compliant Autologous Adoptive Cell Transfer Therapy. Mol. Ther. Methods Clin. Dev. 2017; 4: 178–191.
CrossRef
62. Insel RA, Dunne JL, Atkinson MA et al. Staging presymptomatic type 1 diabetes: a scientific statement of JDRF, the Endocrine Society, and the American Diabetes Association. Diabetes Care 2015; 38: 1964–1974.
CrossRef
63. Bonifacio E, Mathieu C, Nepom GT et al. Rebranding asymptomatic type 1 diabetes: the case for autoimmune beta cell disorder as a pathological and diagnostic entity. Diabetologia 2017; 60: 35–38.
CrossRef
64. Hartl D, Koller B, Mehlhorn AT et al. Quantitative and functional impairment of pulmonary CD4+CD25hi regulatory T cells in pediatric asthma. J. Allergy Clin. Immunol. 2007; 119: 1258–1266.
CrossRef
65. Ling EM, Smith T, Nguyen XD et al. Relation of CD4+CD25+ regulatory T-cell suppression of allergen-driven T-cell activation to atopic status and expression of allergic disease. Lancet 2004; 363: 608–615.
CrossRef
66. Nguyen KD, Vanichsarn C, Fohner A, Nadeau KC. Selective deregulation in chemokine signaling pathways of CD4+CD25(hi)CD127(lo)/(-) regulatory T cells in human allergic asthma. J. Allergy Clin. Immunol. 2009; 123: 933–939(e910).
67. Duan W, So T, Mehta AK, Choi H, Croft M. Inducible CD4+LAP+Foxp3- regulatory T cells suppress allergic inflammation. J. Immunol. 2011; 187: 6499–6507.
CrossRef
68. El Beidaq A, Link CW, Hofmann K et al. In vivo Expansion of Endogenous Regulatory T Cell Populations Induces Long-Term Suppression of Contact Hypersensitivity. J. Immunol. 2016; 197: 1567–1576.
CrossRef
69. Tordesillas L, Mondoulet L, Blazquez AB et al. Epicutaneous immunotherapy induces gastrointestinal LAP(+) regulatory T cells and prevents food-induced anaphylaxis. J. Allergy Clin. Immunol. 2017; 139: 189–201.e184
CrossRef
70. Bonnet B, Vigneron J, Levacher B et al. Low-Dose IL-2 Induces Regulatory T Cell-Mediated Control of Experimental Food Allergy. J. Immunol. 2016; 197: 188–198.
CrossRef
71. Stenger EO, Kazi Z, Lisi E, Gambello MJ, Kishnani P. Immune Tolerance Strategies in Siblings with Infantile Pompe Disease-Advantages for a Preemptive Approach to High-Sustained Antibody Titers. Mol. Genet. Metab. Rep. 2015; 4: 30–34.
CrossRef
72. Banugaria SG, Prater SN, Ng YK et al. The impact of antibodies on clinical outcomes in diseases treated with therapeutic protein: lessons learned from infantile Pompe disease. Genet. Med. 2011; 13: 729–736.
CrossRef
73. Wang J, Lozier J, Johnson G et al. Neutralizing antibodies to therapeutic enzymes: considerations for testing, prevention and treatment. Nat. Biotechnol. 2008; 26: 901–908.
CrossRef
74. Golan L, Goker-Alpan O, Holida M et al. Evaluation of the efficacy and safety of three dosing regimens of agalsidase alfa enzyme replacement therapy in adults with Fabry disease. Drug Des. Devel. Ther. 2015; 9: 3435–3444.
CrossRef
75. Sherman A, Biswas M, Herzog RW. Innovative Approaches for immune tolerance to Factor VIII in the treatment of hemophilia A. Front. Immunol. 2017; 8: 1604.
CrossRef
76. Miao CH. Immunomodulation for inhibitors in hemophilia A: the important role of Treg cells. Expert Rev Hematol. 2010; 3: 469–483.
CrossRef
77. Verma D, Moghimi B, LoDuca PA et al. Oral delivery of bioencapsulated coagulation factor IX prevents inhibitor formation and fatal anaphylaxis in hemophilia B mice. Proc. Natl Acad. Sci. USA 2010; 107: 7101–7106.
CrossRef
78. Sherman A, Su J, Lin S et al. Suppression of inhibitor formation against factor VIII in hemophilia A mice by oral delivery of antigens bioencapsulated in plant cells. Blood 2014; 124(10): 1659–1668.
CrossRef
79. Wang X, Su J, Sherman A, Rogers GL et al. Plant-based oral tolerance to hemophilia therapy employs a complex immune regulatory response including LAP+CD4+ T cells. Blood 2015; 125: 2418–2427.
CrossRef
80. Su J, Sherman A, Doerfler PA et al. Oral delivery of acid alpha glucosidase epitopes expressed in plant chloroplasts suppresses antibody formation in treatment of Pompe mice. Plant Biotechnol. J. 2015; 13(8): 1023–1032.
CrossRef
81. Kim YC, Zhang AH, Su Y et al. Engineered antigen-specific human regulatory T cells: immunosuppression of FVIII-specific T- and B-cell responses. Blood 2015; 125: 1107–1115.
CrossRef
82. Gorzelany JA, de Souza MP. Protein replacement therapies for rare diseases: a breeze for regulatory approval? Sci. Transl. Med. 2013; 5: 178fs110.
CrossRef
83. Vaikunthanathan T, Safinia N, Boardman D, Lechler RI, Lombardi G. Regulatory T cells: tolerance induction in solid organ transplantation. Clin. Exp. Immunol. 2017; 189: 197–210.
CrossRef
84. Safinia N, Vaikunthanathan T, Fraser H et al. Successful expansion of functional and stable regulatory T cells for immunotherapy in liver transplantation. Oncotarget 2016; 7: 7563–7577.
CrossRef
85. Boardman DA, Philippeos C, Fruhwirth GO et al. Expression of a Chimeric Antigen Receptor Specific for Donor HLA Class I Enhances the Potency of Human Regulatory T Cells in Preventing Human Skin Transplant Rejection. Am. J. Transplant. 2017; 17: 931–943.
CrossRef
86. Mohty B, Mohty M. Long-term complications and side effects after allogeneic hematopoietic stem cell transplantation: an update. Blood Cancer J. 2011; 1: e16.
CrossRef
87. Jeffery HC, Braitch MK, Brown S, Oo YH. Clinical Potential of Regulatory T Cell Therapy in Liver Diseases: An Overview and Current Perspectives. Front. Immunol. 2016; 7: 334.
CrossRef
88. Wei HX, Chuang YH, Li B et al. CD4+ CD25+ Foxp3+ regulatory T cells protect against T cell-mediated fulminant hepatitis in a TGF-beta-dependent manner in mice. J. Immunol. 2008; 181: 7221–7229.
CrossRef
89. Chen Y, Sun R, Wu X et al. CD4+CD25+ Regulatory T cells inhibit natural killer cell hepatocytotoxicity of hepatitis B virus transgenic mice via membrane-bound TGF-beta and OX40. J. Innate Immun. 2016; 8: 30–42.
CrossRef
90. Hou X, Song J, Su J et al. CD4(+)Foxp3(+) Tregs protect against innate immune cell-mediated fulminant hepatitis in mice. Mol. Immunol. 2015; 63: 420–427.
CrossRef
91. Hu CC, Jeng WJ, Chen YC et al. Memory Regulatory T cells Increase Only In Inflammatory Phase of Chronic Hepatitis B Infection and Related to Galectin-9/Tim-3 interaction. Sci. Rep. 2017; 7: 15280.
CrossRef
92. Jung MK, Shin EC. Regulatory T cells in hepatitis B and C virus infections. Immune Netw. 2016; 16: 330–336.
CrossRef
93. Trehanpati N, Vyas AK. Immune regulation by T regulatory cells in hepatitis B virus-related inflammation and cancer. Scand J. Immunol. 2017; 85: 175–181.
CrossRef
94. Burzyn D, Kuswanto W, Kolodin D et al. A special population of regulatory T cells potentiates muscle repair. Cell 2013; 155: 1282–1295.
CrossRef
95. Villalta SA, Rosenthal W, Martinez L et al. Regulatory T cells suppress muscle inflammation and injury in muscular dystrophy. Sci. Transl. Med. 2014; 6: 258ra142.
CrossRef
96. Tang Q, Bluestone JA, Kang SM. CD4(+)Foxp3(+) regulatory T cell therapy in transplantation. J. Mol. Cell Biol. 2012; 4: 11–21.
CrossRef
97. Creusot RJ, Battaglia M, Roncarolo MG, Fathman CG. Concise review: cell-based therapies and other non-traditional approaches for Type 1 diabetes. Stem Cells 2016; 34: 809–819.
CrossRef
98. Duggleby R, Danby RD, Madrigal JA, Saudemont A. Clinical grade regulatory CD4(+) T Cells (Tregs): moving toward cellular-based immunomodulatory therapies. Front. Immunol. 2018; 9: 252.
CrossRef
99. Gliwinski M, Iwaszkiewicz-Grzes D, Trzonkowski P. Cell-based therapies with T regulatory cells. BioDrugs 2017; 31: 335–347.
CrossRef
100. Allan SE, Crome SQ, Crellin NK et al. Activation-induced FOXP3 in human T effector cells does not suppress proliferation or cytokine production. Int. Immunol. 2007; 19: 345–354.
CrossRef
101. Nakagawa H, Sido JM, Reyes EE et al. Instability of Helios-deficient Tregs is associated with conversion to a T-effector phenotype and enhanced antitumor immunity. Proc. Natl Acad. Sci. USA 2016; 113: 6248–6253.
CrossRef
102. McClymont SA, Putnam AL, Lee MR et al. Plasticity of human regulatory T cells in healthy subjects and patients with type 1 diabetes. Journal of immunology (Baltimore, Md : 1950) 2011; 186: 3918–3926.
CrossRef
103. Polansky JK, Kretschmer K, Freyer J et al. DNA methylation controls Foxp3 gene expression. Eur J. Immunol. 2008; 38: 1654–1663.
CrossRef
104. Rossetti M, Spreafico R, Saidin S et al. Ex vivo-expanded but not In vitro-induced human regulatory T cells are candidates for cell therapy in autoimmune diseases thanks to stable demethylation of the FOXP3 regulatory T cell-specific demethylated region. J. Immunol. 2015; 194: 113–124.
CrossRef
105. Inomata T, Hua J, Di Zazzo A, Dana R. Impaired function of peripherally induced regulatory T cells in hosts at high risk of graft rejection. Sci. Rep. 2016; 6: 39924.
CrossRef
106. Wei S, Kryczek I, Zou W. Regulatory T-cell compartmentalization and trafficking. Blood 2006; 108: 426–431.
CrossRef
107. Fu H, Kishore M, Gittens B et al. Self-recognition of the endothelium enables regulatory T-cell trafficking and defines the kinetics of immune regulation. Nat. Commun. 2014; 5: 3436.
CrossRef
108. Lee JH, Kang SG, Kim CH. FoxP3+ T cells undergo conventional first switch to lymphoid tissue homing receptors in thymus but accelerated second switch to nonlymphoid tissue homing receptors in secondary lymphoid tissues. J. Immunol. 2007; 178: 301–311.
CrossRef
109. Siegmund K, Feuerer M, Siewert C et al. Migration matters: regulatory T-cell compartmentalization determines suppressive activity In vivo. Blood 2005; 106: 3097–3104.
CrossRef
110. Donnelly C, Dykstra B, Mondal N et al. Optimizing human Treg immunotherapy by Treg subset selection and E-selectin ligand expression. Sci. Rep. 2018; 8: 420.
CrossRef
111. Edozie FC, Nova-Lamperti EA, Povoleri GA et al. Regulatory T-cell therapy in the induction of transplant tolerance: the issue of subpopulations. Transplantation 2014; 98: 370–379.
CrossRef
112. Hartigan-O’Connor DJ, Poon C, Sinclair E, McCune JM. Human CD4+ regulatory T cells express lower levels of the IL-7 receptor alpha chain (CD127), allowing consistent identification and sorting of live cells. J. Immunol. Methods 2007; 319: 41–52.
CrossRef
113. Santegoets SJ, Dijkgraaf EM, Battaglia A et al. Monitoring regulatory T cells in clinical samples: consensus on an essential marker set and gating strategy for regulatory T cell analysis by flow cytometry. Cancer Immunol. Immunother. 2015; 64: 1271–1286.
CrossRef
114. Peters JH, Preijers FW, Woestenenk R, Hilbrands LB, Koenen HJ, Joosten I. Clinical grade Treg: GMP isolation, improvement of purity by CD127 Depletion, Treg expansion, and Treg cryopreservation. PLoS One 2008; 3: e3161.
CrossRef
115. Hoffmann P, Boeld TJ, Eder R et al. Isolation of CD4+CD25+ regulatory T cells for clinical trials. Biol. Blood Marrow Transplant. 2006; 12: 267–274.
CrossRef
116. Golovina T, Mikheeva T, Brusko T, Blazar B, Bluestone J, Riley J. Retinoic acid and rapamycin differentially affect and synergistically promote the ex vivo expansion of natural human T regulatory cells. PLoS One 2011; 6: e15868.
CrossRef
117. Millipore E. SmartFlare RNA detection probes. 2014
118. Schmidl C, Hansmann L, Andreesen R et al. Epigenetic reprogramming of the RORC locus during In vitro expansion is a distinctive feature of human memory but not naive Treg. Eur. J. Immunol. 2011; 41: 1491–1498.
CrossRef
119. Hoffmann P, Boeld TJ, Eder R et al. Loss of FOXP3 expression in natural human CD4+CD25+ regulatory T cells upon repetitive In vitro stimulation. Eur. J. Immunol. 2009; 39: 1088–1097.
CrossRef
120. Schmidl C, Hansmann L, Lassmann T et al. The enhancer and promoter landscape of human regulatory and conventional T-cell subpopulations. Blood 2014; 123: e68–78.
CrossRef
121. Fuhrman CA, Yeh WI, Seay HR et al. Divergent Phenotypes of Human Regulatory T Cells Expressing the Receptors TIGIT and CD226. J. Immunol. 2015; 195: 145–155.
CrossRef
122. Putnam AL, Brusko TM, Lee MR et al. Expansion of human regulatory T-cells from patients with type 1 diabetes. Diabetes 2009; 58: 652–662.
CrossRef
123. Putnam A, Safinia N, Medvec A et al. Clinical grade manufacturing of human alloantigen-reactive regulatory T cells for use in transplantation. American journal of transplantation 2013; 13: 3010–3020.
CrossRef
124. Bluestone J. T1DM Immunotherapy Using CD4+ CD127lo/-CD25+ Polyclonal Tregs. Clin Trials 2011;
125. Hippen KL, Merkel SC, Schirm DK et al. Massive ex vivo expansion of human natural regulatory T cells (T regs) with minimal loss of In vivo functional activity. Science translational medicine 2011; 3: 83ra41-83ra41.
126. Marek N, Bieniaszewska M, Krzystyniak A et al. The time is crucial for ex vivo expansion of T regulatory cells for therapy. Cell Transplant. 2011; 20: 1747–1758.
CrossRef
127. Ye Q, Loisiou M, Levine BL et al. Engineered artificial antigen presenting cells facilitate direct and efficient expansion of tumor infiltrating lymphocytes. J. Transl. Med. 2011; 9: 131.
CrossRef
128. Suhoski MM, Golovina TN, Aqui NA et al. Engineering artificial antigen-presenting cells to express a diverse array of co-stimulatory molecules. Mol. Ther. 2007; 15: 981–988.
<a href=”https://doi.org/10.1038/mt.sj.6300134
129. Maus MV, Thomas AK, Leonard DG et al. Ex vivo expansion of polyclonal and antigen-specific cytotoxic T lymphocytes by artificial APCs expressing ligands for the T-cell receptor, CD28 and 4-1BB. Nat. Biotechnol. 2002; 20: 143–148.
CrossRef
130. Golovina TN, Mikheeva T, Suhoski MM et al. CD28 costimulation is essential for human T regulatory expansion and function. J. Immunol. 2008; 181: 2855–2868.
CrossRef
131. Putnam AL, Brusko TM, Lee MR et al. Expansion of human regulatory T-cells from patients with type 1 diabetes. Diabetes 2009; 58: 652–662.
CrossRef
132. Seay HR, Putnam AL, Cserny J et al. Expansion of Human Tregs from Cryopreserved Umbilical Cord Blood for GMP-Compliant Autologous Adoptive Cell Transfer Therapy. Mol. Ther. Methods Clin. Dev. 2017; 4: 178–191.
CrossRef
133. Fan Z, Spencer JA, Lu Y et al. In vivo tracking of ‘color-coded’ effector, natural and induced regulatory T cells in the allograft response. Nat. Med. 2010; 16: 718–722.
CrossRef
134. Tang Q, Lee K. Regulatory T-cell therapy for transplantation: how many cells do we need? Curr. Opin. Organ Transplant. 2012; 17: 349–354.
CrossRef
135. Kinsey GR, Sharma R, Huang L et al. Regulatory T cells suppress innate immunity in kidney ischemia-reperfusion injury. J. Am. Soc. Nephrol. 2009; 20: 1744–1753.
CrossRef
136. Antunes I, Kassiotis G. Suppression of innate immune pathology by regulatory T cells during Influenza A virus infection of immunodeficient mice. J Virol 2010; 84: 12564–12575.
CrossRef
137. Riley JL, June CH, Blazar BR. Human T regulatory cell therapy: take a billion or so and call me in the morning. Immunity 2009; 30: 656–665.
CrossRef
138. Giang S, La Cava A. Regulatory T cells in SLE biology and use in treatment. Curr. Rheumatol. Rep. 2016; 18: 67.
https://doi.org/10.1007/s11926-016-0616-6″ rel=”noopener” target=”_blank”>CrossRef
139. Sackstein R, Schatton T, Barthel SR. T-lymphocyte homing: an underappreciated yet critical hurdle for successful cancer immunotherapy. Lab. Invest. 2017; 97(6): 669-697.
CrossRef
140. Brusko T, Bluestone J. Regulatory T cells directed to the site of the action. Proc. Natl Acad. Sci. USA 2009; 106: 20553–20554.
CrossRef
141. Brusko TM, Koya RC, Zhu S et al. Human antigen-specific regulatory T cells generated by T cell receptor gene transfer. PLoS One 2010; 5: e11726.
https://doi.org/10.1371/journal.pone.0011726″ rel=”noopener” target=”_blank”>CrossRef
142. Putnam AL, Safinia N, Medvec A et al. Clinical grade manufacturing of human alloantigen-reactive regulatory T cells for use in transplantation. Am. J. Transplant. 2013; 13: 3010–3020.
CrossRef
143. Tarbell KV, Yamazaki S, Olson K, Toy P, Steinman RM. CD25+ CD4+ T cells, expanded with dendritic cells presenting a single autoantigenic peptide, suppress autoimmune diabetes. J. Exp. Med. 2004; 199: 1467–1477.
CrossRef
144. Eugster A, Lindner A, Catani M et al. High diversity in the TCR repertoire of GAD65 autoantigen-specific human CD4+ T cells. J. Immunol. 2015; 194: 2531–2538.
CrossRef
145. Babon JA, DeNicola ME, Blodgett DM et al. Analysis of self-antigen specificity of islet-infiltrating T cells from human donors with type 1 diabetes. Nat. Med. 2016; 22: 1482–1487.
CrossRef
146. Delong T, Wiles TA, Baker RL et al. Pathogenic CD4 T cells in type 1 diabetes recognize epitopes formed by peptide fusion. Science 2016; 351: 711–714.
CrossRef
147. Kracht MJ, van Lummel M, Nikolic T et al. Autoimmunity against a defective ribosomal insulin gene product in type 1 diabetes. Nat. Med. 2017; 23: 501–507.
CrossRef
148. Porter DL, Levine BL, Kalos M, Bagg A, June CH. Chimeric antigen receptor-modified T cells in chronic lymphoid leukemia. N. Engl. J. Med. 2011; 365: 725–733.
CrossRef
149. Grupp SA, Kalos M, Barrett D et al. Chimeric antigen receptor-modified T cells for acute lymphoid leukemia. N. Engl. J. Med. 2013; 368: 1509–1518.
CrossRef
150. Maude SL, Frey N, Shaw PA et al. Chimeric antigen receptor T cells for sustained remissions in leukemia. N. Engl. J. Med. 2014; 371: 1507–1517.
CrossRef
151. Porter DL, Hwang WT, Frey NV et al. Chimeric antigen receptor T cells persist and induce sustained remissions in relapsed refractory chronic lymphocytic leukemia. Sci. Transl. Med. 2015; 7: 303ra139.
CrossRef
152. Fraietta JA, Beckwith KA, Patel PR et al. Ibrutinib enhances chimeric antigen receptor T-cell engraftment and efficacy in leukemia. Blood 2016; 127: 1117–1127.
CrossRef
153. Chakraborty R, Rooney C, Dotti G, Savoldo B. Changes in chemokine receptor expression of regulatory T cells after ex vivo culture. J. Immunother. 2012; 35: 329–336.
CrossRef
154. Yurchenko E, Tritt M, Hay V et al. CCR5-dependent homing of naturally occurring CD4+ regulatory T cells to sites of Leishmania major infection favors pathogen persistence. J. Exp. Med. 2006; 203: 2451–2460.
CrossRef
155. Wang CR, Liu MF. Regulation of CCR5 expression and MIP-1alpha production in CD4+ T cells from patients with rheumatoid arthritis. Clin. Exp. Immunol. 2003; 132: 371–378.
CrossRef
156. Baeke F, Korf H, Overbergh L et al. The vitamin D analog, TX527, promotes a human CD4+CD25highCD127low regulatory T cell profile and induces a migratory signature specific for homing to sites of inflammation. J. Immunol. 2011; 186: 132–142.
CrossRef
157. Curiel TJ, Coukos G, Zou L et al. Specific recruitment of regulatory T cells in ovarian carcinoma fosters immune privilege and predicts reduced survival. Nat. Med. 2004; 10: 942–949.
CrossRef
158. Yuan Q, Bromley SK, Means TK et al. CCR4-dependent regulatory T cell function in inflammatory bowel disease. J. Exp. Med. 2007; 204: 1327–1334.
CrossRef
159. Trzonkowski P, Bacchetta R, Battaglia M et al. Hurdles in therapy with regulatory T cells. Sci. Transl. Med. 2015;7:304ps318
CrossRef
160. Dawson NAJ, Vent-Schmidt J, Levings MK. Engineered Tolerance: Tailoring Development, Function, and Antigen-Specificity of Regulatory T Cells. Front. Immunol. 2017; 8: 1460.
CrossRef
161. Jones BS, Lamb LS, Goldman F, Di Stasi A. Improving the safety of cell therapy products by suicide gene transfer. Front. Pharmacol. 2014; 5: 254.
CrossRef
162. Gargett T, Brown MP. The inducible caspase-9 suicide gene system as a “safety switch” to limit on-target, off-tumor toxicities of chimeric antigen receptor T cells. Front. Pharmacol. 2014;5; 235: .
CrossRef
163. Lozano E, Dominguez-Villar M, Kuchroo V, Hafler DA. The TIGIT/CD226 axis regulates human T cell function. J. Immunol. 2012; 188: 3869–3875.
CrossRef
164. Burn GL, Svensson L, Sanchez-Blanco C, Saini M, Cope AP. Why is PTPN22 a good candidate susceptibility gene for autoimmune disease? FEBS Lett 2011; 585: 3689–3698.
CrossRef
165. Passerini L, Bacchetta R. Forkhead-Box-P3 Gene transfer in human CD4(+) T conventional cells for the generation of stable and efficient regulatory t cells, suitable for immune modulatory therapy. Front. Immunol. 2017; 8: 1282.
CrossRef
166. Makadia HK, Siegel SJ. Poly Lactic-co-glycolic acid (PLGA) as biodegradable controlled drug delivery carrier. Polymers-Basel 2011; 3: 1377–1397.
CrossRef
167. Cohen S, Yoshioka T, Lucarelli M, Hwang LH, Langer R. Controlled delivery systems for proteins based on poly(lactic/glycolic acid) microspheres. Pharm. Res. 1991; 8: 713–720.
CrossRef
168. Kasturi SP, Sachaphibulkij K, Roy K. Covalent conjugation of polyethyleneimine on biodegradable microparticles for delivery of plasmid DNA vaccines. Biomaterials 2005; 26: 6375–6385.
CrossRef
169. Elamanchili P, Diwan M, Cao M, Samuel J. Characterization of poly(D,L-lactic-co-glycolic acid) based nanoparticulate system for enhanced delivery of antigens to dendritic cells. Vaccine 2004; 22: 2406–2412.
CrossRef
170. Jain S, O’Hagan DT, Singh M. The long-term potential of biodegradable poly(lactide-co-glycolide) microparticles as the next-generation vaccine adjuvant. Expert Rev. Vaccines 2011; 10: 1731–1742.
CrossRef
171. Jhunjhunwala S, Balmert SC, Raimondi G et al. Controlled release formulations of IL-2, TGF-beta 1 and rapamycin for the induction of regulatory T cells. J. Controlled Release 2012; 159: 78–84.
CrossRef
172. Jiang WL, Gupta RK, Deshpande MC, Schwendeman SP. Biodegradable poly(lactic-co-glycolic acid) microparticles for injectable delivery of vaccine antigens. Adv. Drug Deliver. Rev. 2005; 57: 391–410.
CrossRef
173. Stephan MT, Moon JJ, Um SH, Bershteyn A, Irvine DJ. Therapeutic cell engineering with surface-conjugated synthetic nanoparticles. Nat. Med. 2010;16:1035-U1135
CrossRef
174. Esensten JH, Bluestone JA, Lim WA. Engineering therapeutic t cells: from synthetic biology to clinical trials. Annu. Rev. Pathol. 2017; 12: 305–330.
CrossRef
175. Tey SK, Bollard CM, Heslop HE. Adoptive T-cell transfer in cancer immunotherapy. Immunol. Cell. Biol. 2006; 84: 281–289.
CrossRef
176. Rosenberg SA, Restifo NP, Yang JC, Morgan RA, Dudley ME. Adoptive cell transfer: a clinical path to effective cancer immunotherapy. Nat. Rev. Cancer 2008; 8: 299–308.
CrossRef
177. Papaioannou NE, Beniata OV, Vitsos P, Tsitsilonis O, Samara P. Harnessing the immune system to improve cancer therapy. Ann. Transl. Med. 2016; 4: 261.
CrossRef
178. Brun V, Bastian H, Neveu V, Foussat A. Clinical grade production of IL-10 producing regulatory Tr1 lymphocytes for cell therapy of chronic inflammatory diseases. Int. Immunopharmacol. 2009; 9: 609–613.
CrossRef
179. Golab K, Grose R, Trzonkowski P et al. Utilization of leukapheresis and CD4 positive selection in Treg isolation and the ex-vivo expansion for a clinical application in transplantation and autoimmune disorders. Oncotarget 2016; 7: 79474–79484.
CrossRef
180. Taylor PA, Lees CJ, Blazar BR. The infusion of ex vivo activated and expanded CD4(+)CD25(+) immune regulatory cells inhibits graft-versus-host disease lethality. Blood 2002; 99: 3493–3499.
CrossRef
181. Zhou P, Borojevic R, Streutker C, Snider D, Liang H, Croitoru K. Expression of dual TCR on DO11.10 T cells allows for ovalbumin-induced oral tolerance to prevent T cell-mediated colitis directed against unrelated enteric bacterial antigens. J. Immunol. 2004; 172: 1515–1523.
CrossRef
182. Tarbell KV, Petit L, Zuo Xe et al. Dendritic cell-expanded, islet-specific CD4+ CD25+ CD62L+ regulatory T cells restore normoglycemia in diabetic NOD mice. J. Exp. Med. 2007; 204: 191–201.
CrossRef
183. Masteller EL, Warner MR, Tang Q et al. Expansion of functional endogenous antigen-specific CD4+CD25+ regulatory T cells from nonobese diabetic mice. J. Immunol. 2005; 175: 3053–3059.
CrossRef
184. Trenado A, Sudres M, Tang Q et al. Ex vivo-expanded CD4+CD25+ immunoregulatory T cells prevent graft-versus-host-disease by inhibiting activation/differentiation of pathogenic T cells. J. Immunol. 2006; 176: 1266–1273.
CrossRef
185. Golshayan D, Jiang S, Tsang J et al. In vitro-expanded donor alloantigen-specific CD4+CD25+ regulatory T cells promote experimental transplantation tolerance. Blood 2007; 109: 827–835.
CrossRef
186. Tsang JY, Tanriver Y, Jiang S et al. Conferring indirect allospecificity on CD4+CD25+ Tregs by TCR gene transfer favors transplantation tolerance in mice. J Clin Invest 2008; 118: 3619–3628.
CrossRef
187. Joffre O, Santolaria T, Calise D et al. Prevention of acute and chronic allograft rejection with CD4+CD25+Foxp3+ regulatory T lymphocytes. Nat. Med. 2008; 14: 88–92.
CrossRef
188. Stephens LA, Malpass KH, Anderton SM. Curing CNS autoimmune disease with myelin-reactive Foxp3+ Treg. Eur J. Immunol. 2009; 39: 1108–1117.
CrossRef
189. Wright GP, Notley CA, Xue SA et al. Adoptive therapy with redirected primary regulatory T cells results in antigen-specific suppression of arthritis. Proc. Natl Acad. Sci. USA 2009; 106: 19078–19083.
CrossRef
190. Sagoo P, Ali N, Garg G et al. Human regulatory T cells with alloantigen specificity are more potent inhibitors of alloimmune skin graft damage than polyclonal regulatory T cells. Sci. Transl. Med. 2011;3:83ra42
CrossRef
191. Mekala DJ, Geiger TL. Immunotherapy of autoimmune encephalomyelitis with redirected CD4+CD25+ T lymphocytes. Blood 2005; 105: 2090–2092.
CrossRef
192. Mekala DJ, Alli RS, Geiger TL. IL-10-dependent infectious tolerance after the treatment of experimental allergic encephalomyelitis with redirected CD4+CD25+ T lymphocytes. Proc. Natl Acad. Sci. USA 2005; 102: 11817–11822.
CrossRef
193. Elinav E, Waks T, Eshhar Z. Redirection of regulatory T cells with predetermined specificity for the treatment of experimental colitis in mice. Gastroenterology 2008; 134: 2014–2024.
CrossRef
194. Fransson M, Piras E, Burman J et al. CAR/FoxP3-engineered T regulatory cells target the CNS and suppress EAE upon intranasal delivery. J Neuroinflammation 2012; 9: 112.
CrossRef
195. Blat D, Zigmond E, Alteber Z, Waks T, Eshhar Z. Suppression of murine colitis and its associated cancer by carcinoembryonic antigen-specific regulatory T cells. Mol. Ther. 2014; 22: 1018–1028.
CrossRef
196. MacDonald KG, Hoeppli RE, Huang Q et al. Alloantigen-specific regulatory T cells generated with a chimeric antigen receptor. J. Clin. Invest. 2016; 126: 1413–1424.
CrossRef
197. Noyan F, Zimmermann K, Hardtke-Wolenski M et al. Prevention of Allograft Rejection by Use of Regulatory T Cells With an MHC-Specific Chimeric Antigen Receptor. Am. J. Transplant. 2017; 17: 917–930.
CrossRef
198. Pierini A, Iliopoulou BP, Peiris H et al. T cells expressing chimeric antigen receptor promote immune tolerance. J. CI. Insight 2017;2 2(20).
CrossRef
Affiliations
Gregory P Marshall II1#, Judit Cserny2#, Daniel J Perry2, Wen-I Yeh2, Howard R Seay2, Ahmed G Elsayed2,3, Amanda L Posgai2 and Todd M Brusko1,2*
1 OneVax LLC, Sid Martin Biotechnology Institute, Alachua, Florida, USA
2 Department of Pathology, Immunology, and Laboratory Medicine, University of Florida Diabetes Institute, Gainesville, Florida, USA
3 Department of Microbiology and Immunology, Faculty of Medicine, Mansoura University, Egypt
# Co-first author with equal contributions to the manuscript.
* Author for correspondence: tbrusko@ufl.edu
This work is licensed under a Creative Commons Attribution- NonCommercial – NoDerivatives 4.0 International License</