The Importance of Collection, Processing & Biopreservation Best Practices in Determining CAR-T Starting Material Quality
Cell Gene Therapy Insights 2018; 4(4), 327-336.
10.18609/cgti.2018.032
The approval of several CAR-T cell therapies highlights the ongoing transition of cell-based therapeutics from clinical trial to commercialization. This transition stage is difficult at the best of times, encompassing a shift from small- to large-scale drug production while maintaining product safety and efficacy. Cell therapy presents a unique challenge not extant to traditional medical treatments. Living cells embody an intrinsic variability of response and function that can impact their efficacy in a patient. It is important that pharmaceutical companies take pains to optimize their production workflow from the beginning, to ensure an effective and reliable product. The need for high-quality starting material is crucial to success, since the number of healthy cells in leukapheresis-derived starting material directly impacts the efficacy of the final product. In this article, we examine the processes that ensure optimal quality of the material that forms the basis of CAR-T therapies.
Submitted for Peer Review: Mar 22 2018 Published: May 14 2018
Cell therapy research and clinical development has accelerated sharply in the past two decades. Once limited to bone marrow transplants, the cell therapy field is now poised to bring about significant advances in a number of different clinical indications. Some of the most notable advances are being made in the field of immunotherapy. Chimeric antigen receptor (CAR)-T cell therapy is a rapidly emerging cancer immunotherapy that has been generating great excitement in the medical field. Clinical trials have demonstrated the remarkable effectiveness of CAR-T cell therapy in the treatment of acute lymphocytic leukemia (ALL) and certain types of B-cell lymphoma [1]. With the first few CAR-T therapies now approved for clinical use, and more in clinical trial stage, pharmaceutical companies such as Novartis and Kite Pharma (Gilead) are gearing up for the large-scale manufacture of these products [2]. Manufacture of CAR-T cell products faces unique challenges due to the intrinsic variability of both leukapheresis-derived precursor materials, and processing methods for the living cells that comprise the therapeutic product. Any drug product approved for human use must meet specified targets of safety and efficacy; more than that, each dose should have the same potency in every patient every time. The importance of product consistency cannot be overstated. Such consistency can only be achieved by developing specific guidelines for starting material quality. To realize commercial success, manufacturers will need to adopt best practices, by standardizing optimal methods of leukapheresis collection, T cell selection and isolation procedures, and biopreservation methods.
Donor Selection & Sourcing
Even the most sophisticated cellular therapy has to start somewhere. It is important to remember that the biological material used to manufacture such a therapy begins with a human donor, a fact that introduces variability from the start. While automation is driving many manufacturing processes to become more closely defined, the quality of cell therapy starting material remains neither well defined nor standardized. Part of the problem is rooted in availability; indeed, the limited availability of cell and gene therapy starting material as these therapeutics edge closer to large-scale manufacturing is a pressing one [3,4]. For European biopharmaceutical companies, regulations prohibiting monetary reimbursement of donors for biological products only exacerbates the problem. European companies often source leukapheresis and other blood-related products from the USA, where donor reimbursement regulations are less restrictive. However, sourcing from overseas carries an additional risk of a negative impact on starting material due to long shipping distances, customs delays or inadequate cold chain management [4,5].
Another factor that can affect starting material quality is donor sourcing. CAR-T cell therapy currently relies primarily on autologous treatments sourced from the patient, in order to match individual cancer subtypes and avoid immunogenicity issues. While these individualized therapies are a boon to the patient, it is important to remember that the immune system of a cancer patient is often impacted by their disease and can look quite different from that of a healthy donor. Just as genetic factors will vary with each donor, factors such as disease state, medical history, age, demographics and lifestyle will also vary, and any or all of these factors can influence the composition of the material being collected [6–8]. Most leukemia patients have too many immature white cells in their blood [6], making it difficult to obtain sufficient mature T cells for CAR-T cell modification and expansion. Tumor cells also release factors that let them escape detection and destruction by suppressing the immune system [9]. This can affect the type and number of T cell subtypes present in a leukapheresis collection, adding to the risk of manufacturing failure [10]. Since donor sourcing for autologous therapy carries intrinsic challenges, CAR-T manufacturers must compensate by optimizing T cell isolation techniques during both the leukapheresis procedure and isolation methods that follow [4,11]. CAR-T manufacturers should also set quality criteria for acceptable viable cell counts and immune cell subpopulation ratios for leukapheresis material.
Because it is difficult to obtain sufficient high-quality precursor material from patients with weakened or impacted immune systems [12,13], leukapheresis material from patient-matched healthy donors is frequently used for process development of autologous CAR-T cell therapies. Many small biotech start-ups working on novel CAR-T therapies rely on local hospitals and academic leukapheresis labs for obtaining starting material. Large-scale production and delivery of a pharmaceutical product, however, means working on a precise schedule that matches patient availability at numerous clinical centers across multiple distribution points. Reliance on a small local donor pool, while adequate for early phase investigations and development, is not workable for large-scale process development, for which a steady supply of starting material from healthy donors is needed (Figure 1).
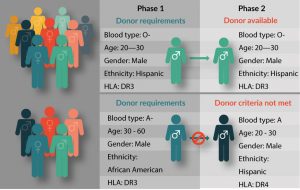
Figure 1: A Recallable Donor Database Supports Clinical Trial Success.
Assuring consistent availability of high-quality precursor material for large-scale distribution means assuring a large, reliable donor database that meets all FDA and cGMP/cGTP requirements. Biotechnology companies that provide human blood products should have access to AABB-accredited donor centers, and IRB-approved informed donors from a well-characterized database of diverse genetic backgrounds. The ability to request repeat collection from high-value donors is another important consideration. A high-value donor is one who is healthy and reliable, consistently responds well to immune cell mobilization, and provides large volume leukapheresis collection with high therapeutic cell counts [5,14]. Leukapheresis product quality and consistency impacts quality of the final product [15,16], and establishing a good donor relationship increases the likelihood that these high-value ‘superdonors’ will be amenable to repeat collections [16]. Leukapheresis providers with a large, recallable donor pool have a better chance of sourcing starting material from the right donors at the right time, helping researchers to match target patient populations during process development. There are as yet too few biological supply companies with a donor database large enough to reliably supply leukapheresis products for the burgeoning field of commercialized CAR-T cell products, and many in the industry are aware that this supply issue urgently needs to be addressed [12,17]. A model for rigorously controlled, GMP/GTP-compliant leukapheresis collection best practices has been outlined by Burger et al. [15].
Since donor supply is an ongoing challenge to autologous CAR-T cell therapy, researchers have been obliged to consider, and more recently develop, allogeneic options [18–20]. These ‘off-the-shelf’ CAR-T therapies seek to rely on healthy donors for sourcing clinical grade leukapheresis products. Access to recallable donors is particularly useful to allogeneic CAR-T clinical studies, where patient characteristics must be matched as closely as possible. A pool of recallable donors can be used to supplement the eligible donor pool for a given patient, and help ensure that both quality criteria and supply volume can reliably be met. In a study co-authored by researchers from the National Institute of Health and the National Cancer Institute [21], HLA-matched siblings were used as donors for allogeneic CAR-T cell clinical trial to treat patients with B-cell malignancies. The trial, one of the first of its kind, met with highly encouraging results, with 8 of 20 patients achieving remission. Because allogeneic cells carry a significantly higher risk of immunogenicity, this new CAR-T approach is mitigated upon engineering donor cells to be CAR-modified like their autologous counterparts, but also T cell receptor (TCR) deficient to avoid immunogenicity issues [22]. Thus far the technique looks promising; none of the patients in the Brudno study developed new-onset graft-versus-host disease, which is a common complication with allogeneic donors. It is to be hoped that the capacity to use healthy donor cells for CAR-T therapy will bring down costs while significantly alleviating donor supply issues, allowing these therapies to be manufactured on a large scale and with ‘off-the-shelf’ readiness.
Collection & Processing Methods
While the donor is probably the most significant source of starting material variability, collection methods are also a contributing factor. During leukapheresis, the donor’s blood is collected and separated into various components. The desired blood components, such as plasma, platelets, red blood cells and white blood cells are separated out, usually by centrifugation. The remaining blood components are then returned to the donor’s circulation. Collecting leukapheresis material from a donor requires a trained operator, preferably with substantial experience in the procedure. Leukapheresis training and collection protocols vary considerably across clinical sites, as does the leukapheresis collection equipment itself. Even leukapheresis centers with highly trained personnel tend to experience process drift over time. Standardized operating procedures and GMP/GTP compliant collection protocols will help limit variability and guarantee consistent quality.
Standardizing leukapheresis collection equipment can be difficult since collection sites are not always part of the manufacturing process. Pharmaceutical companies that wish to ensure that leukapheresis centers associated with their product are using optimal and standardized equipment, may be tasked with the cost and responsibility of sponsoring the transition to blood collection systems that support best practices for CAR-T cell product manufacturing. The Spectra Optia® Apheresis System (Terumo BCT, Lakewood, CO, USA), is currently favored in the industry as a state-of-the-art cell collection platform [23], and most, though not all, modern hospitals and clinics utilize this equipment. However, while individual hospitals can provide high-quality leukapheresis material, large-scale expansion of CAR-T products will necessitate standardization across multiple clinical sites. A more efficient path forward may be to partner with commercial suppliers of human blood products with their own network of accredited leukapheresis collection centers. CAR-T manufacturers using a commercial supplier have the freedom to set their own quality requirements regarding acceptable leukocyte cell counts and purity. Since commercial suppliers utilize a network of clinics, they can often access a robust donor database that includes a wide selection of disease state products to facilitate patient matching. They can also assure that equipment, protocols and training are fully standardized across all clinical sites.
Collection protocols are yet another factor impacting leukapheresis product variability. Leukapheresis is a specific type of apheresis focused on separating white blood cells out from whole blood. This is the method used for collecting T cells for CAR-T cell therapy. As CAR-T cell development geared up to larger, multicenter clinical trials, it became evident that a major issue facing developers was the insufficient number of target cells present in some collections; whether through insufficient collection volume, insufficient white blood cell counts or significant contamination with unwanted cell populations. Chemotherapy, which can compromise the immune system, is a major culprit in low white blood cells counts [24], since CAR-T clinical trial eligibility is thus far limited to those patients who have failed to respond to standard chemotherapy regimens. The risk of CAR-T therapy manufacturing failure is directly related to the number of T cells (as measured by absolute lymphocyte count) found in the leukapheresis product [25]. There is also preliminary evidence that the ratio of specific T cell subpopulations affects downstream efficacy. One study from CHOP/University of Pennsylvania indicated that the number of early lineage T cells present in starting material is directly related to the efficacy of therapeutic T cell expansion and persistence once they are infused back into the patient [26]. Given the importance of initial lymphocyte counts, CAR-T cell manufacturers generally utilize collection protocols that enrich for the mononuclear cell (MNC) layer. In many cases, the monocyte component of the collected material, which is used for dendritic cell therapies, can be selectively depleted to enrich for the T cell population in the leukapheresis product.
Biopreservation Methods
Unless collected leukapheresis material is going to be used immediately, it must be either stored until it can be processed or transported to the CAR-T manufacturing site. Cell therapy products are highly temperature-sensitive, and even short excursions from their optimal storage temperature can negatively impact the downstream efficacy of the final product. As soon as leukapheresis material is collected, steps must be taken to ensure that cells remain viable and functional [27,28]. As CAR-T products move into the global manufacturing and distribution arena, these ‘living drugs’ will be exposed to longer shipping times and a higher risk of inadvertent temperature changes. Best practice for shipping and storage of cell therapy material, whether it be starting material or the finished product, must include taking steps to protect cold chain integrity (Figure 2) [29].
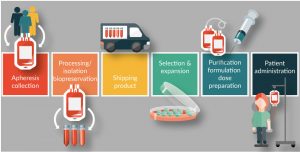
Figure 2: Apheresis Cell Therapy Commercialization.
In addition to temperature optimization, stability of the starting material may be optimized with utilization of biopreservation media. An effective, well-designed biopreservation media mitigates risk by extending the shelf life of, and optimizing the quality of, cell therapy starting material [30,31]. The shelf life of freshly isolated leukapheresis products at room temperature is minimal; ranging anywhere from 30 minutes to a few hours, depending on the desired application [32]. Consequently, cell therapy starting material is handled in one of two ways. If the collection and treatment sites are located in the same clinic or close by one another, freshly isolated leukapheresis products can be processed within a short period of time. Once initial processing is complete, target cells may either be stored for further processing or administered to the patient.
Alternatively, if cellular starting material is to be transported off-site, it will either be prepared for cryopreservation and long-term storage, or reformulated for short-term storage at 2–8°C. This precaution protects cells from degradation, ensuring they retain maximum efficacy at the delivery site. Storage at 2–8°C has the benefit of slowing down cellular metabolism and reducing the physiological stress that can lead to functional damage or cell death. However, hypothermic temperature alone may not provide optimized protection, and cells may experience stresses from cold storage that can also lead to cell damage and cell death. An extensive body of research [32–34] has focused on developing optimal biopreservation and cryopreservation media for various cell types, under different storage conditions. Examples of such media engineered for low-temperature biopreservation include the intracellular-like formulations HypoThermosol FRS and CryoStor, which are designed in contrast to isotonic media formulated for normothermic conditions. Hypothermic biopreservation media should ideally protect cells from osmotic stress and free radical damage during exposure to hypothermic temperatures [35].
Cryopreservation is considered a practical necessity for many in the cellular therapy field, solving logistical issues such as scheduling flexibility, long-term storage, biobanking cells for future CAR-T manufacturing runs or treatment, and shipping to multiple clinical sites. Cryopreservation seeks to preserve living cells in a stable state, free from the ravages of metabolic processes or chemical reactions. While traditional cryopreservation methods have been adequate in this endeavor, cryopreservation is not without risk or room for optimization. The stress of freeze-thaw cycles on cells can lead to damage and loss of yield/viability/function via necrosis, apoptosis and secondary necrosis. Well-controlled freezing and thawing rates, and consistent cryopreservation protocols that incorporate fully-defined, optimized, intracellular-like GMP freezing media have demonstrated preservation of starting material viable recovery and function [36]. The commercialization of CAR-T cell therapies will require standardization of donor qualification parameters, collection processes and cold chain management. Setting standardized criteria for starting material quality parameters and handling practices will minimize product variability and protect downstream efficacy.
Translational Insight
Leukapheresis products are the starting material for today’s CAR-T cell therapies. Therapeutic potency is directly impacted by factors such as donor selection criteria, collection procedures, and cellular isolation and biopreservation methods. Specific guidelines and supporting data for starting material quality, combined with better donor supply logistics will help minimize variability and optimize downstream clinical outcomes.
Financial & competing interests disclosure
Lou Juliano and George Eastwood are employees of HemaCare Corporation. Aby J Mathew and Todd Berard are employees of BioLife Solutions, Inc. Writing assistance from APEX Think was utilized in the production of this manuscript.
References
1. Maude SL, Teachey DT, Porter DL, Grupp SA. CD19-targeted chimeric antigen receptor T-cell therapy for acute lymphoblastic leukemia. Blood 2015; 125: 4017–23.
CrossRef
2. Stanton D. Gilead aims to expand CAR-T manufacturing capabilities post Kite acquisition. BioPharma-Reporter Aug 2017.
3. Walker A, Johnson R. Chimeric Antigen Receptor Therapy in Haematology and Oncology: Current Successes and Challenges. Biochem. Soc. Trans. 2016; 44, 329–32.
CrossRef
4. Levine BL, Miskin J, Wonnacott K, Christopher Keir C. Global Manufacturing of CAR T Cell Therapy. Mol. Ther. Methods Clin. Dev. 2017; 17(4): 92–101.
https://doi.org/10.1016/j.omtm.2016.12.006″ rel=”noopener” target=”_blank”>CrossRef
5. Larkin M, Ecot A, Learn CA, Lachs M. The challenges of manufacturing and distributing CAR-T therapies. Pharmafocus 2017 Nov.
6. Abrahamsen JF, Stamnesfet S, Liseth K, Hervig T, Bruserud O. Large-volume leukapheresis yields more viable CD34+cells and colony-forming units than normal-volume leukapheresis, especially in patients who mobilize low numbers of CD34+cells. Transfusion 2005; 45(2): 248-53.
CrossRef
7. Pinho MP, Barbuto JAM. Systemic alterations in T cell subpopulations of breast cancer patients. Abstracts: CRI-CIMT-EATI-AACR Inaugural International Cancer Immunotherapy Conference: Translating Science into Survival; September 16–19 2015; New York, NY, USA.
8. Saavedra D, Garcia B, Lage A. T Cell Subpopulations in Healthy Elderly and Lung Cancer Patients: Insights from Cuban Studies. Front. Immunol. 2017; 8: 146.
CrossRef
9. Arber DA. Acute Myeloid Leukemia. Atlas of Bone Marrow Pathology. Springer, New York, NY, USA. 2018; 173–191.
CrossRef
10. Oldreive CE, Skowronska A, Davies NJ et al. T-cell number and subtype influence the disease course of primary chronic lymphocytic leukaemia xenografts in alymphoid mice. Dis. Model Mech. 2015; 8: 1401–12.
CrossRef
11. Fesnak A, O’Doherty U. Clinical Development and Manufacture of Chimeric Antigen Receptor T cells and the Role of Leukapheresis. Eur. Oncol. Haematol. 2017; 13(1): 28–34.
CrossRef
12. Sumen C. Understanding Today’s CAR-T Cell Therapy Manufacturing Challenges. PCT (Hitachi). 2017 May.
13. Off to the CAR T Races: Bringing CAR T-Cell Therapies to Cancer Patients. ASH Clinical News. 2017 November.
14. Hosing C, Saliba RM, Hamerschlak M. Peripheral blood stem cell yield calculated using preapheresis absolute CD34+ cell count, peripheral blood volume processed, and donor body weight accurately predicts actual yield at multiple centers. Transfusion. 2013 October.
15. Burger S, Juliano L, Wang W. Cellular Raw Material Collection in Cell Therapy: Critical Determinant of Product Quality. Drug Discov. World 2014; 15(3): 29–34.
16. Juliano L. Defining best practice in allogeneic cell therapy and autologous process development donor supply and management. Phacilitate Cell Gene Therapy Forum 2018, Miami, FL, USA.
17. Vaz W, Shirazian A, Smith S et al. Sourcing Clinical-Grade Human Tissue: Considerations for Supporting Cell Therapy Development and Production. BioProcess International. 2018 March.
18. Fernandez CR. The First Off-the-Shelf CAR-T Cancer Therapy Has Entered the Clinic. 2017 June.
19. De Almeida M. Novartis Gains Access to Celyad’s allogenic CAR-T cell patents. From the Clinic (Novartis) 2017 Feb.
20. CRISPR Therapeutics Presents Positive Data on Allogeneic CRISPR-based CAR-T Cell Therapies at AACR. CRISPR Therapeutics. 2018.
21. Brudno JN, Somerville RPT, Shi V et al. Allogeneic T Cells That Express an Anti-CD19 Chimeric Antigen Receptor Induce Remissions of B-Cell Malignancies That Progress After Allogeneic Hematopoietic Stem-Cell Transplantation Without Causing Graft-Versus-Host Disease [published online January 25, 2016]. J. Clin. Oncol. 2016; 34(10): 1112–21.
CrossRef
22. First US Patent Covering Allogeneic Chimeric Antigen Receptor T Cells (“CAR-T”) Modified to Reduce Immunogenicity is Awarded to Celyad. Businesss Wire. 2015 October.
23. Kim J, Joseph R, Matevosyan K, Sarode R. Comparison of Spectra Optia and COBE Spectra apheresis systems’ performances for red blood cell exchange procedures. Transfus. Apher. Sci. 2016; 55(3): 368–70.
CrossRef
24. Verma R, Foster RE, Horgan K et al. Lymphocyte depletion and repopulation after chemotherapy for primary breast cancer. Breast Cancer Res. 2016; 18: 10.
CrossRef
25. Singh N, Perazzelli J, Grupp SA, M. Barrett DM. Early memory phenotypes drive T cell proliferation in patients with pediatric malignancies. Sci. Transl. Med. 2016; 8(320): 320ra3.
https://doi.org/10.1126/scitranslmed.aad5222″ rel=”noopener” target=”_blank”>CrossRef
26. Chao JL, Savage PA. Unlocking the Complexities of Tumor-Associated Regulatory T Cells. J. Immunol. 2018; 200(2); 415–21.
CrossRef
27. Riviere I. Overcoming the Key Challenges in Delivering Cell and Gene Therapies to Patients: A View from the Front Line. Cell Gene Ther. Insights 2016; 2(2), 249–54.
CrossRef
28. Abazari A, Hawkins BJ, Clarke DM, Mathew AJ. Biopreservation Best Practices: A Cornerstone in the Supply Chain of Cell-based Therapies – MSC Model Case Study. Cell Gene Ther. Insights. 2017; 2017; 3(10), 853–71.
29. Correia C, Koshkin A, Carido M et al. Effective Hypothermic Storage of Human Pluripotent Stem Cell-Derived Cardiomyocytes Compatible with Global Distribution of Cells for Clinical Applications and Toxicology Testing. Stem Cells Trans. Med. 2016; 5: 658–69.
CrossRef
30. Ramos T, Wang W, Okhovat J, McPherson G, Burger S. Human-derived raw materials: Controlled, consistent collections enable successful manufacturing of cell-based regenerative medicine products. J. Immunol. 2014; 192: 69.15.
31. Hawkins BJ, Abazari A, Mathew AJ. Biopreservation Best Practices for regenerative medicine GMP manufacturing & focus on optimized biopreservation media. Cell Gene Ther. Insights 2017; 3: 345–58.
CrossRef
32. Angel S, Briesen H, Oh Y, Baller MK, Zimmermann H, Germann A. Toward Optimal Cryopreservation and Storage for Achievement of High Cell Recovery and Maintenance of Cell Viability and T Cell Functionality. Biopreservation Biobanking 2016; 14(6): 539–46.
<a href=”https://doi.org/10.1089/bio.2016.0046
33. Ivanics T, Bergquist JR, Liu G et al. Patient-derived xenograft cryopreservation and reanimation outcomes are dependent on cryoprotectant type. Lab. Invest. 2018.
CrossRef
34. Gniadek TJ, Garritsen HSP, Stroncek D, Szczepiorkowski ZM, McKenna DH. Optimal Storage Conditions for Apheresis Research (OSCAR): A Biomedical Excellence for Safer Transfusion (BEST) Collaborative study. Transfusion 2017; 58(2): 461–9.
CrossRef
35. BioLife Solutions CryoStor® Cell Freeze Media Highlighted in Mayo Clinic/MD Anderson Journal Article on Preservation of Patient-Derived Xenografts for Cancer Research. PRNewswire/BioLifeSolutions, Inc. Mar 2018.
36. Wang W, Hexom T, Nucho L, Eastwood G. Cryopreserved Leukopaks Maintain Cell Viability and Functionality: A Solution for Cell Therapy Logistics. International Society for Cellular Therapy Annual Meeting, Las Vegas NV, USA, 2015 May.
Affiliations
Lou Juliano1*, George Eastwood1, Todd Berard2 & Aby J Mathew2
1 HemaCare Corporation, 15350 Sherman Way, Suite 423, Van Nuys, CA 91406, USA
2 BioLife Solutions Inc., 3303 Monte Villa Parkway, Suite 310, Bothell, WA 98021, USA
* Author for correspondence: ljuliano@hemacare.com
This work is licensed under a Creative Commons Attribution- NonCommercial – NoDerivatives 4.0 International License</