Advances and challenges in umbilical cord blood and tissue bioprocessing: procurement and storage
Cell Gene Therapy Insights 2017; 3(6), 489-508.
10.18609/cgti.2017.042
Umbilical cord tissue and blood is banked to complement the rapidly advancing field of tissue engineering and regenerative medicine for both autologous and allogeneic therapeutic applications. Whilst many problems concerning the use of the hematopoietic and multipotential mesenchymal stromal cells contained therein may be addressed through the future development of GMP-compliant manufacturing strategies, collection and bioprocessing of these tissues can be optimised in the present to maximise clinical outcomes. In this review, we describe current procurement, processing and storage approaches for umbilical cord blood and tissue; current challenges and how these may be met to augment translation and use of therapeutics harnessing their derivatives.
Cord blood and tissue banking is increasingly popular on account of the stem cells they contain and the clinical research associated with such material advances. Umbilical cord blood (UCB) is a source of hematopoietic stem cells (HSCs; hereafter referred to as UCB-HSC) and is clinically applied for the reconstitution of hematopoiesis [1,2]. Umbilical cord tissue (UCT) contains mesenchymal stem cells (hereafter referred to as UCT-MSC) that have been widely investigated for applications in tissue restoration and repair, and the treatment of immune-mediated disorders [3,4]. UCB is defined as the blood that remains in the umbilical cord and placenta following neonatal delivery, and UCT as the cord itself. Both tissues are procured immediately after birth in a process not deleterious to mother or child. In fact, banking of these tissues may confer future health benefits to the corresponding child or immediate family as they potentially comprise an exact or partial human leukocyte antigen (HLA) match, reducing complications such as graft-versus-host disease (GvHD) upon transplantation. Additionally, both tissues may be applied in allogeneic models; UCT-hMSC are immune evasive and donor-recipient mismatches at some HLA loci are well tolerated in UCB transplantation [5].
The potential applications of UCB-HSC and UCT-MSC in both autologous and allogeneic therapeutics has encouraged the coexistence, sometimes within the same organization, of two tissue storage models [6]. Parents may choose to publicly bank their UCB for use for any patient, or store privately in the event of disease in the immediate family for which UCB transplantation is indicated. Over 750,000 UCB units are thought to be banked publicly worldwide, with over 4 million banked in private family storage arrangements [7]. No storage data is available for UCT, in part because UCT-MSC has no current clinical application, and consequently is not publicly banked. There are, however, a considerable number of clinical trials concerning the application of UCT-MSC, creating demand for UCT processing strategies that main the viability, efficacy and safety of any derived therapeutic (Table 1). In this review, we offer insight into how the tissue bioprocessing of today may be tailored to ensure the safety and efficacy of the UCB- and UCT-derived cell therapies of the future.
Table 1: Summary of the first 15 open clinical trials, sorted by relevance, found by searching ‘umbilical mesenchymal stem cells’ as an intervention at www.clinicaltrials.gov at the time of writing (n = 40). | ||||
---|---|---|---|---|
Clinical indication | Donor type | Phase | Overview | Identifier |
Asthma | Allogeneic | Phase I/II | Safety and early efficacy study of intra-nasal administration of MSC trophic factors | NCT02192736 |
Autism spectrum disorder (ASD) | Allogeneic | Phase I | Safety study of administration of UCT-MSC every 2 months in children with ASD | NCT03099239 |
Allogeneic | Phase I/II | Safety and early efficacy study of administration of UCT-MSC every 3 months for 1 year in children with ASD | NCT02192749 | |
Multiple sclerosis | Allogeneic | Phase I/II | Safety and early efficacy study of effect of administration of UCT-MSC daily for 7 days in MS patients | NCT02034188 |
Rheumatoid arthritis | Allogeneic | Phase I/II | Safety and early efficacy study on the effect of treatment with UCT-MSC daily for 5 days to induce remission | NCT01985464 |
Allogeneic | Phase I | Safety and early efficacy study of administration of UCT-MSC weekly for 4 weeks | NCT02643823 | |
Aplastic anemia | Allogeneic | Phase I | Safety and early efficacy study of UCT-MSC administration | NCT03055078 |
Hepatic cirrhosis | Allogeneic | Phase I | Safety and early efficacy study of weekly UCT-MSC administration for 4 weeks | NCT02652351 |
Spinal cord injury | Allogeneic | Phase III | Safety and efficacy study of intrathecal administration of UCT-MSC | NCT02481440 |
Cerebral hemorrhage | Allogeneic | Phase I | Safety and early efficacy study of weekly UCT-MSC administration for 4 weeks | NCT02283879 |
Type 1 diabetes | Allogeneic | Phase III | Efficacy study of UCT-MSC treatment of patients with Type 1 diabetes | NCT02763423 |
Systemic lupus erythematosus | Allogeneic | Phase II | Efficacy study of administration of UCT-MSC | NCT02633163 |
Nonunion fracture | Allogeneic | Early Phase I | Comparative study of treatment of non-union fractures with UCT-, bone marrow- or adipose tissue derived MSC | NCT02307435 |
Umbilical cord blood bioprocessing
Transplantation of HSC is an established treatment for reconstitution of hematopoiesis in numerous disease states [2]. These include myelodysplastic syndromes [8,9], leukemias [10,11], lymphomas [12], multiple sclerosis [13], metabolic diseases [14] and numerous disorders of blood cell proliferation, including sickle cell disease and Fanconi’s anemia (Table 2) [1,15]. UCB provides a viable alternative to other source tissues, including peripheral blood (PB) and bone marrow (BM) [16]. Although most HSC transplantations are currently sourced from PB, challenges persist that may be resolved through the use of UCB. For example, HSC extraction from PB by apheresis after administration of hematopoietic growth factors has known side effects in pediatric donors including perivascular pain, emesis, hypotension, urticaria, numbness, chest pain, facial flushing and hypocalcemia. The lower intracorporeal blood volume of children is believed to result in this reduced safety profile, with a complication rate of 6% recently reported in such patients [17,18]. Significant immune hemolysis upon transplantation has also been reported as a result of minor ABO blood group system incompatibility between donor and recipient [19]. Additionally, the long duration of apheresis (~4 hours), which must be supervised by a health care professional (HCP), increases healthcare costs.
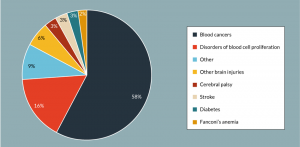
Figure 1: Representation of the clinical target of all open trials investigating UCB as a clinical intervention at the time of writing (n= 139). Clinical targets included in the ‘other’ category include HIV infection, low birth weight and various types of hemorrhage.
Bone marrow, obtained in an invasive and painful procedure, has commonly been used as a source of HSC [20]. The functional characteristics of HSC are not constant throughout life; they exhibit reduced proliferative potential and myeloid-biased differentiation output with aging. This reduced capacity for lymphoid differentiation is believed to account for the decline in immune function in the elderly, and consequently HSC from aged donors administered for hematopoietic reconstitution may result in poorer patient outcomes than HSC from younger donors, such as the neonate population from which UCB-HSC are derived [21,22].
UCB offers an allogeneic source of HSC in lieu of PB and BM, and has been employed in clinical trials for the treatment of a range of indications including blood cancers, diabetes, stroke and Fanconi’s anemia (Figure 1 & Table 2). In fact, since the first successful transplant in 1988 for the treatment of Fanconi’s anaemia [1], it is estimated that more than 35,000 UCB transplants have been performed worldwide [7].
Table 2: Summary of the first 15 open clinical trials, sorted by relevance, found by searching for ‘umbilical cord blood’ as an intervention at www.clinicaltrials.gov at the time of writing (n =139). | ||||
---|---|---|---|---|
Clinical indication | Donor type | Phase | Overview | Identifier |
Cerebral palsy | Allogeneic | Not provided | Efficacy study of UCB administration in children with CP under non-myeloblative immunosuppression | NCT01639404 |
Autologous | Phase II | Safety study of UCB administration in children with CP | NCT01147653 | |
Allogeneic | Phase I | Safety study of sibling UCB transplant in children with CP | NCT02599207 | |
Allogeneic | Phase II | Efficacy study of UCB administration in children with CP under non-myeloblative immunosuppression | NCT01528436 | |
Global development delay | Allogeneic | Not provided | Efficacy study of UCB administration in children with Global Development Delay under non-myeloblative immunosuppression | NCT01601158 |
Ischemic stroke | Allogeneic | Phase I | Safety study of single UCB unit transplantation, matched by ABO/Rh, within 3-10 days of acute stroke | NCT02397018 |
Allogeneic | Phase I | Safety study of UCB transplantation and mannitol within 72 hours of stroke | NCT02433509 | |
Allogeneic | Phase II | Efficacy study of single UCB unit transplantation, matched by ABO/Rh and ethnicity, within 3-10 days of acute stroke | NCT03004976 | |
Autism spectrum disorder | Autologous | Phase I | Safety study of single UCB unit transplantation in children with ASD | NCT02176317 |
Infertility | Allogeneic | Not provided | Efficacy study of the treatment of infertility caused by sever intrauterine adhesions or endometrial dysplasia by UCB-derived MSCs on a collagen scaffold | NCT02313415 |
Congenital pediatric disorders | Allogeneic | Not provided | Safety study of UCB transplantation for congenital paediatric disorders, esp. wrt incidence of GvHD | NCT00950846 |
Immunodeficiency | Allogeneic | Phase II | Efficacy of intrabone infusion of UCB to improve haematopoietic reconstitution | NCT01711788 |
Hypoplastic left heart syndrome (HLHS) | Autologous | Phase I | Safety and feasibility study of intraventricular administration of UCB in children with HLHS | NCT01883076 |
Neonatal encephalopathy | Autologous | Phase I | Safety and feasibility of UCB transplantation within 72 hours postpartum | NCT02256618 |
Type 1 diabetes | Allogeneic | Phase I/II | Isolation, ex vivo expansion and transplantation of regulatory T cells from UCB | NCT02932826 |
Procurement, processing & storage of UCB
UCB procurement
As for most tissue donation, donors of UCB, regardless of storage model, must provide informed consent prior to collection, and undergo infectious disease screening between 7 days before and 7 days after delivery (Figure 2, stage A). Units are always procured postpartum, but may be collected before or after separation of the placenta from the uterus. These methods are termed in and ex utero procurement respectively (Figure 2, stage C) [23]. Additionally, UCB procurement is not limited by birth type; units are routinely collected following both induced and non-induced vaginal and cesarean births. For all approaches, the blood supply from the umbilical cord to the neonate is interrupted by postpartum clamping following birth, and the residual cord blood extracted by venipuncture. In the UK, the Human Tissue Authority (HTA) recommends ex utero procurement of UCB [24]; in contrast, in utero collection is widely favoured elsewhere, including the USA and Western Europe, due to the significantly greater sample volumes and total nucleated cell counts (TNCs) achieved [25]. This may be due to the fact that, during the third stage of labor, the uterus continues to contract, aiding the expulsion of blood [26].
Impact of obstetric practices on UCB quality
The final volume and total nucleated cell count (TNC) of UCB collected is partially dependent on uncontrollable obstetric factors, such as maternal age [27], gestational period [28,29], placental weight [30], number of prior live births [31,32], delivery type [33-35], maternal smoking [36], cord length [28,37], ethnicity [38], maternal preeclampsia [39,40], fetal distress [34], birth weight [28,41], and possibly neonate sex [27]. However, birth and procurement approaches, under clinician and maternal control, may also impact UCB unit quality. For example, the timing of cord clamping directly impacts the volume of UCB collected [42] and the pre-venipuncture preparation of the cord impacts UCB unit contamination [43,44].
Delayed cord clamping, classed as interruption of the blood supply between cord and infant after more than one minute postpartum, is recommended by the World Health Organisation (WHO) [45]; extended placental transfusion following delivery improves term infant iron status for up to 6 months and reduces the risk of maternal postpartum hemorrhage (Figure 2, stage B) [26,46]. Additionally, delayed cord clamping reduces the risk of intraventricular hemorrhage, late-onset sepsis and necrotizing enterocolitis in pre-term neonates [47]. The loss of blood flow as a result of delayed cord clamping, temperature changes and other factors cause vascular occlusion, which in turn triggers coagulation of entrapped blood [48]. The volume of UCB units collected following delayed cord clamping are significantly reduced as a result, although some tissue banks advise that UCB collection is still viable if the cord is clamped after no more than 3 minutes. Similarly, those procured following expectant versus actively managed labors (whereby prophylactic uterotonics are administered to hasten delivery of the extraembryonic tissues) are also of smaller volume [49].
UCB is contained within the aseptic, closed system comprising baby, placenta and cord, and is drained into a sterile container for subsequent processing. Microbial contamination is therefore not present in either UCB (in the absence of vertically transmitted infections) or storage materials; in fact, contaminants may only be introduced at the point of procurement during which UCB is briefly exposed to the cord exterior. The birth environment is inherently non-sterile because, particularly during vaginal births, the epithelial amniotic membrane is in contact with vaginal and colon-derived fluids. Microbes transferred via these fluids then access the cord interior through the procurement venipuncture. The risk of microbial contamination of UCB through venipuncture should be reduced through the use of antimicrobial surgical swabs at the puncture site prior to drainage; hence HCP compliance with tissue procurement instructions is vital in ensuring the suitability of UCB units for clinical application [43].
Transport of UCB
Following procurement, UCB units and any other samples collected are placed in transport packaging (Figure 2, stage E). During sample transit, environmental conditions critical to the maintenance of cell viability, such as the partial pressures of CO2 and O2, temperature and pH are largely uncontrolled and are therefore reliant on external conditions and the duration of transit. However, packaging may be optimized to both meet regulatory shipping requirements and mediate environmental changes. For example, thermally insulating materials, such as polystyrene, may be used in sample packaging, which also isolates UCB from the influence of light. Phase change materials (PCMs), that have a high latent heat capacity, incorporated within gel packs, can be placed in transport boxes, to further augment temperature buffering [50]. Additionally, temperature recording apparatus may be included in transport boxes, and samples may be transported in refrigerated vehicles or packaging to retard microbial growth in transit, and maintain cell viability [51]. Additionally, cooling of the external environment may have limited success for samples stored in thermally insulated packaging; the authors suggest that suitable ‘inside out’ cooling technologies that may be enclosed in transport boxes are lacking and should be developed.
In the authors’ experience, logistical factors between the site of procurement and storage can impinge on the quality of a cord blood unit. Interestingly, private cord blood banking is prohibited in some countries in favor of public banking models, but export of samples for overseas processing and storage is sometimes permitted. On average, the procurement volume of private units originating from such countries is smaller than from other regions [Unpublished data]; we speculate that this may reflect the reservations of HCPs regarding the ethics of private cord blood banking. Furthermore, extended transit, as may be caused by geographical and local customs/legal requirements, is associated with lower white blood cell counts and HSC viability [Unpublished data].
Bioprocessing of UCB for cryopreservation
UCB processing may incorporate automated or operator-based, and whole blood or volume-reduced approaches (Figure 2, stage G onwards). Economic, safety and quality considerations inform the technologies employed in a given paradigm. A balance must be sought between the comparatively reduced storage costs and the greater processing time required for volume reduced versus whole blood cord units [52]. Additionally, volume-reduced transplants confer improved patient outcomes, such as a reduction in ABO incompatibility alloreactivity and dimethyl sulfoxide (DMSO) toxicity (Figure 2, stage H) [53].
In volume reduction protocols, the red blood cell and plasma fractions of units are largely depleted. This approach has been adopted by many banks worldwide, including public organisations such as the NHS Cord Blood Bank (UK). In summary, incoming units are separated by centrifugation or filtration and plasma, red blood cell and HSC-containing ‘buffy coat’ fractions are separated using either semi-automated devices such as the Macopress Smart Evo (Macopharma, France), Optipress II (Fenwal, USA) and CellEffic (Kaneka, Japan) products, or fully automated, enclosed systems (such as the Sepax™ (Biosafe, USA) or AutoXpress® (Cesca Therapeutics, USA) [49,54]. Cryoprotectant is added to the buffy coat fraction, which is stored in vapor phase N2. Sedimentation agents such as pentastarch [55] and hydroxyethyl starch (HES) [56] may also be used to enhance red blood cell removal. Fully or semi-automated systems may offer advantages with respect to standardization, necessary to ensure the safety and efficacy of UCB-derived cell therapies, and allow UCB processing to take place in ungraded environments. In whole blood storage protocols, the complete unit is cryopreserved following cryoprotectant addition with no additional processing steps [57].
Challenges in the clinical application of UCB
Use of UCB for haematopoietic reconstitution was first reported by Ende et al., for the treatment of acute lymphoblastic leukemia, in 1972 [58]. Unfortunately, clinical use of UCB is limited by the availability of human leucocyte antigen (HLA)-matched donors, although mismatches at one or two loci are well tolerated [5]. Approximately 30% of all recipients are matched with an HLA-identical donor through worldwide registries; around 75% of Caucasians [59], but only 17% of other ethnicities find a suitable donor [60].
Low hematopoietic progenitor yields per unit present a yet more significant challenge for the clinical use of UCB, and mean that only low weight patients, such as children, are eligible for transplant of single units [61]. Low cell doses are also associated with increased engraftment times, prolonged immunodeficiency and greater healthcare burdens [62]. Double UCB transplantation, an alternative treatment regimen that increases the number of HSC transplanted, has been widely reported to improve clinical outcomes [63], but is associated with greater acquisition costs. However, one notable study by Labopin et al., in which Markov analysis was used to evaluate the costs and outcomes of single and double UCB transplantation in France, reported the latter as more cost-effective [64]. Alternatively, ex vivo expansion of UCB-HSC may increase available cell doses, and manufacturing strategies to produce advanced therapy medicinal products (ATMP) are under investigation [65]. Current UCB therapies are considered minimally manipulated products by regulators [66,67].
Umbilical cord tissue bioprocessing
UCT is banked to preserve UCT-MSC, which offer different clinical potentials to UCB-HSC. The identification of clonogenic fibroblast-like cells in bone marrow by Friedenstein et al. in 1970 [68] is oft viewed as the dawn of modern MSC research. MSC have subsequently been widely investigated for applications in wound healing and various pathologies, utilizing their multilineage plasticity and immunomodulatory functions [5,69]; at the time of writing this review, there are approximately 2600 clinical trials involving MSC, of which 40 utilized UCT-derived MSC (www.clinicaltrials.gov; search terms: “mesen*” AND “cell”, “MSC”, “umb*” AND “mesen*”). Although MSC reside in virtually all postnatal tissues [70], they have been frequently sourced from bone marrow. Major drawbacks associated with bone marrow sources include the invasiveness of aspiration, influence of donor age on the functional characteristics of MSC and their paucity within this tissue. In comparison, UCT is considered clinical waste, is relatively MSC dense, and is sourced from neonates [71]. Other clinically useful properties of MSC include the lack of expression of MHC-II (major histocompatibility complex II) and co-stimulatory molecules CD80 and CD86, conferring hypoimmunogenicity. Hence, MSC derived from suitable sources and subjected to appropriate bioprocessing may also be harnessed for ‘off-the-shelf’ allogeneic therapeutics [72].
Challenges in procurement and storage of UCT
UCT is procured following neonate delivery and transported for processing in transport solution. Following neonate delivery and UCB procurement the cord is severed from the placenta, disinfected, and placed in a sterile container (Figure 3, stages A–E). Tissue viability and sterility are impacted by the same transit, packaging and environmental factors as described previously for UCB (Figure 3, stage F). Upon arrival at a processing laboratory, cord tissue processing is typically performed using the ‘slice and dice’ method (Figure 3, stage J) [73]; briefly, UCT is disinfected, rinsed, minced and added to cryoprotectant before controlled rate freezing and long-term storage in vapor-phase N2 (Figure 3, stages G–M). Although tissue procurement is relatively uncontrolled due to the clinical demands of the birth environment, the authors recognize that key aspects of industry practice may be improved to optimize UCT collection and storage [74].
Microbial contamination of UCT
The quality of banked cord tissue may be considered in terms of sterility, cell yield, functionality and anticipated safety for clinical application. Since UCT is maintained in aseptic conditions throughout transit and processing, microbial contamination may only be introduced at the point of procurement as a result of contact with the ex utero environment. The collection method used may influence the probability of microbial contamination; we have found that in utero procurement can be associated with UCT contamination rates approximately three times greater than ex utero counterparts [Unpublished data]. Additionally, microbial species found on in utero procured samples are overwhelmingly of faecal origin; this is likely due to extended proximity to excreta in the birth environment [Unpublished data]. The course, type, and duration of birth may be reasonably expected to affect the probability of microbial contamination but are not controllable to the UCT procurer. However, the impact of these factors and hence contact with bodily fluids may be mitigated by thorough disinfection of all samples using surgical swabs (Figure 3, stage D).
Additionally, we have also found that there may be a significant relationship between sample transit duration and the incidence of contaminated cord tissue (Figure 3, stage F) [Unpublished data]. It is postulated that this may be explained by increased microbial growth with increasing transit times. Longer incubation times may also encourage the formation of biofilm communities that are not easily subsequently eliminated; biofilms are often recalcitrant to the antibiotic and biocidal measures fatal to planktonic bacteria [75].
Yield & functionality of MSC content
The yield and functionality of MSC subsequently isolated from UCT is affected by tissue bioprocessing methods. For example, intra- and inter- cord variability in MSC content should be considered with respect to tissue procurement. An optimal cell donor cannot be selected in autologous paradigms, but strategic tissue sampling is possible. Lim et al. investigated the yield and functionality of UCT-hMSC isolated from tissue segments excised from distinct cord regions. Fetal and maternal segment-derived MSC had greater viability, lower doubling times and higher differentiation potentials than middle cord-derived MSC [76]. Hence, selective tissue resection from the ends of umbilical cords may improve UCT-hMSC characteristics, but further evidence is necessary to support any change in collection practices.
In allogeneic models, donor MSC are expanded for off-the-shelf supply, and both inter- and intra-cord sampling comprise controllable variables. Allogeneic donor criteria must incorporate infectious disease screening and demographic factors; studies concerning the impact of donor characteristics are limited, but reports indicate that gestational diabetes mellitus and maternal age of ˃29 years impact the functional characteristics of UCT-hMSC, and hence should form exclusion criteria [77,78].
Safety considerations in bioprocessing of UCT
The cryoprotectants and choice of transport/wash solutions used for UCT bioprocessing directly contribute to patient outcomes. For example, UCT is often transported in penicillin-containing solutions despite the well-documented allergenicity of β-lactam antibiotics. In fact, penicillin allergy affects up to 8% of patients, and complications can be fatal [79]. In the opinion of the authors, the use of antibiotic-treated tissues in cell therapy manufacture will present increased challenges in gaining regulatory approval.
Additionally, both cryoprotectant and cryopreservation media choice pose safety concerns. The most commonly used cryoprotectant, dimethyl sulfoxide (DMSO), is toxic at the molar concentrations used and at temperatures greater than 4°C [71,80]. In fact, a plethora of adverse reactions have been reported from DMSO exposure in humans and other mammals, including some following post-thaw infusion of washed cells in clinical trials, where up to 50% of patients were affected [81,82]. The allo- or xeno-geneic components of serum-containing cryopreservation media, believed to supply nutrients in the post-thaw period, are notoriously difficult to remove and may trigger immunogenic responses [83].
Challenges in isolation of MSC from UCT
UCT banking has the potential to meet clinical and commercial MSC demands with the maturation of bioprocessing and expansion technologies. Unlike the UCB-HSC, UCT-MSC must always be expanded ex vivo to supply clinically relevant cell doses. Although a critical area for research and development activities, the scope of this review comprises current progress and challenges in the procurement and processing of UCT for cryopreservation. Readers are referred to the following reviews for insight regarding the ex vivo expansion of MSC [84-86].
Isolation of MSC from UCT has been described in a number of publications [87-89], but there is no consensus regarding an optimal approach that maximizes cell yield, viability and population homogeneity. UCT-MSC are isolated either by enzymatic disaggregation of cord tissue or the selective migration of MSC from cultured explants. Collagenase, hyaluronidase and trypsin are commonly used in digestion protocols [90,91], either alone or in combination, and different explant sizes and explant-substrate adhesion modes have been investigated [92]. Only one report has emerged in which a combination of both methods was investigated [93].
MSC are found throughout UCT, which may be stored as whole minced tissue, with a lack of consensus amongst investigators as to whether UCT-MSC isolated from different anatomical regions are equivalent [94,95]. However, MSC are most commonly isolated from Wharton’s jelly or the perivascular region [96,97]. Although these anatomical regions contain approximately 75% of UCT-MSC and this approach may reduce the number of contaminating epithelial and endothelial cells in early passage UCT-MSC cultures, incorporating dissection of an inherently variable tissue into a closed, automated process according to best practice, remains problematic [98,99]. Since the macroscale dimensions of individual cords differ, separation of distinct tissues must be conducted by a skilled operator, and to meet cGMP standards, manual bioprocessing must be housed in a class A biosafety cabinet installed in a Class B room [68,100].
Preservation, shipping & contamination management of UCB and UCT
Contamination management strategies in UCB and UCT banking must be devised with care to maximize the clinical utility of these tissues. We propose that two main approaches should be employed to reduce the incidence of contamination: management of HCP practices to avoid initial microbial inoculation of tissue, and ablation of microbial growth during transit.
Owing to the inflated contamination rates observed in in utero procured UCT, ex utero procurement of these tissues is recommended to ensure the safety of derived UCT-MSC. Alternatively, if HCPs strongly favor the in utero collection method, the sample should be taken to a designated clean area after UCB procurement for thorough disinfection according to the processing facility’s protocol, which should incorporate a combined disinfection approach such as treatment with both chlorhexidine and alcohol, rather than alcohol alone, since this combination results in greater microbial kill [101]. Whilst the formation of biofilms on both in and ex vivo soft tissues is widely acknowledged in microbiology, to the authors’ knowledge, no investigators have attempted to detect biofilm matrix on contaminated UCT to date. Such results could be instrumental in refining current industry practices.
Penicillin-containing solutions have frequently been added to tissue transport solutions to ablate bacterial growth during transit. Whilst the impact of growth during transit is acknowledged to be large, penicillin is a common allergen and treated tissue is unlikely to find allogeneic uses. The most frequently encountered contaminant species on UCT and UCB are mesophilic, and moderate cooling during transit is suggested to extend population doubling times as an alternative [102,103]. The increased expense of courier services using refrigerated versus ambient vehicles means that refrigeration inside sample packaging is more economically viable. This may be achieved through addition of cooling devices to sample packaging in conjunction with thermally insulating packaging.
Other safety concerns impacting the utility of UCB and UCT in the clinic include cryopreservation strategies, and whole versus volume -reduced bioprocessing of UCB. The success of cryopreservation is dependent on cryopreservation medium and the method of cooling. Controlled rate freezing of DMSO-infused tissues is preferred throughout the tissue banking industry; vitrification of UCT has previously been attempted to the detriment of the UCT-MSC contained within [104]. The toxicity of DMSO to both cells and man means that the lowest effective concentration should be used and post-thaw recovery optimized. Concentrations of 10% (v/v) DMSO are often used, but increasing evidence suggests that this can be reduced to 2.5% (v/v), with benefits for the functional characteristics of UCB-HSC and UCT-MSC, and reducing the risk of infusional toxicity of DMSO [105,106]. Current practices achieve DMSO removal by washing with isotonic salt solutions and centrifugation, or using automated cell washing devices [107], but these introduce mechanical and osmotic stresses that can result in significant cell loss [71].
Slow-cooling cell injury can be further precluded through inclusion of polysaccharides such as trelahose [108], sugar alcohols, hydroxyethyl starch (HES) [109] and dextran in cryopreservation media [110]. DMSO readily permeates cell membranes and impedes extracellular ice formation by depressing the freezing point of water, and polysaccharides are thought to assist by confounding the formation of intracellular ice [111]. The addition of polysaccharide solutions also reduces the concentration of DMSO necessary for successful cryopreservation [106,112]. Hence, we recommend that the minimum concentration of DMSO required, validated with respect to optimal post-thaw cell recovery, in conjunction with the nontoxic additives previously listed, be used to cryopreserve UCT and UCB for translation/clinical application.
Although UCT may be stored in a saline/DMSO solution, serum-containing culture media are also widely used in cryopreservation media. Although the Federal Drug Administration (FDA) (USA) permits cell therapy manufacture with cGMP-compliant sera [113], xeno- and allogeneic antigens present may be hyperimmunogenic to patients, and zoonotic, viral and prion transmission is possible. Chemically defined, serum-free medium (SFM) minimizes lot-to-lot variability in cell products, but culture substrates coated with exogenous proteins are frequently needed to aid adherence. Fortunately, successful xeno- and serum-free MSC culture has now been reported, and should be harnessed in cryopreservation media for tissue banking [114].
Volume reduction of UCB is commonplace because it reduces per unit storage costs and improves transplantation outcomes. At present, there are no known clinical uses for the RBC fraction of cord blood, and RBC lysate generated during cryopreservation and thawing can impact kidney function in recipients [61]. Alloreactivity of whole blood UCB units may also present where donor and recipient have differing blood groups, regardless of HLA type [115]. Consequently, volume reduction is recommended in UCB processing for maximal transplant success.
Translational insight
Ultimately, effective bioprocess development is dependent upon an intimate knowledge of critical quality attributes (CQAs) of the final product and intended clinical application. Transplantation of UCB-MSC is a standard therapy for numerous diseases, but no UCT-MSC therapeutics currently exist. This is reflected in the fact that, at the time of writing, there are approximately 139 active clinical trials of UCB interventions, but just 40 employing UCT-MSC (Figures 1 & 4). Despite this, UCT-MSCs are being investigated as a therapy in clinical indications such as GvHD, hepatic cirrhosis, myocardial infarction and myopathies, spinal cord injury, arthritis, multiple sclerosis, and stroke [71,116]. A ‘chicken and egg’ paradigm exists in cell therapy research – bioprocess strategies are required to facilitate ATMP development, but clinical direction is needed to define CQAs of such bioprocesses. Clinical trials are suggested as the best indicators of clinically important parameters in tissue banking of UCB and UCT (Tables 1 & 2).
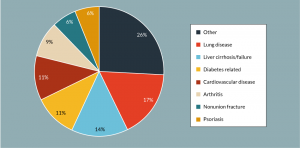
Figure 4: Representation of the clinical target of all open trials investigating UCT-MSC as an intervention at the time of writing (n = 40).
In the absence of scalable manufacturing technology, the demand for high UCT-MSC and UCB-HSC doses can be tackled through the adoption of optimal bioprocess and clinical approaches that seek to preserve both maximal cell numbers and engraftment potentials. The number of HSC administered has been increased using the scale out approach of double UCB transplantation, maintaining the ‘minimally manipulated’ regulatory status of the treatment [117]. Reduced intensity patient conditioning prior to transplantation [118,119], direct intra-bone injection [120,121] and co-transplantation of UCB with MSC for their immunoprotective function [122,123], have all been utilized to maximize engraftment. We suggest that the number of UCT-hMSC sampled during UCT collection may be optimized through selective procurement, where practicable, of equal sized tissue segments from the fetal and placental ends of the cord.
A consensus regarding an optimal method for the isolation of UCT-MSC is yet to emerge, with scant data available to assess the merits of explant and enzymatic digestion methods. Thus, comparison studies of UCT-MSC extracted by each method are contrasted, according to their kinetics and the defining criteria outlined by the International Society for Cellular Therapy (ISCT) are required for effective translation [124].
Conclusion
To complement the body of work that aims to elucidate clinical uses of UCB and UCT-MSC, bioprocessing strategies that are optimal with respect to cell functionality, patient outcomes and cost must be developed. In this review, we argue that UCB volume reduction, antibiotic-free bioprocesses, low DMSO in cryopreservation media, and improved antimicrobial strategies may aid the readiness of UCB and UCT banked in the present for incorporation into the cell therapeutics of the future.
financial & competing interests disclosure
LP, SM, KS & BC wish to acknowledge their involvement with Biovault Technical Ltd. The authors have no other relevant financial involvement with an organization or entity with a financial interest in or financial conflict with the subject matter or materials discussed in the manuscript. No writing assistance was utilized in the production of this manuscript.
References
1. Gluckman E, Broxmeyer HE, Auerbach AD et al. Hematopoietic reconstitution in a patient with Fanconi’s anemia by means of umbilical-cord blood from an HLA-identical sibling. N. Engl. J. Med. 1989; 321:1174–1178.
CrossRef
2. Laughlin MJ, Barker J, Bambach B et al. Hematopoietic engraftment and survival in adult recipients of umbilical-cord blood from unrelated donors. N Engl J Med 2001; 344:1815–1822.
CrossRef
3. Hudson KD, Bonassar LJ. Hypoxic Expansion of Human Mesenchymal Stem Cells Enhances 3D Maturation of Tissue Engineered Intervertebral Discs. Tissue Eng. Part A 2016; 23: 293-300.
4. Li WJ, Tuli R, Okafor C et al. A three-dimensional nanofibrous scaffold for cartilage tissue engineering using human mesenchymal stem cells. Biomaterials 2005; 26:599–609.
CrossRef
5. Ankrum J a, Ong JF, Karp JM. Mesenchymal stem cells: immune evasive, not immune privileged. Nat. Biotechnol. 2014; 32:252–260.
CrossRef
6. Hauskeller C, Beltrame L. The hybrid bioeconomy of umbilical cord blood banking: Re-examining the narrative of opposition between public and private services. Biosocieties 2015; 11:1745–8552.
7. Ballen KK, Verter F, Kurtzberg J. Umbilical cord blood donation: public or private? Bone Marrow Transplant. 2015; 50:1271–1278.
CrossRef
8. Eapen M, Klein JP, Sanz GF et al. Effect of donor-recipient HLA matching at HLA A, B, C, and DRB1 on outcomes after umbilical-cord blood transplantation for leukaemia and myelodysplastic syndrome: A retrospective analysis. Lancet Oncol. 2011; 12:1214–1221.
CrossRef
9. Ooi J, Iseki T, Takahashi S et al. Unrelated cord blood transplantation for adult patients with advanced myelodysplastic syndrome. Blood 2003; 101:4711–4713.
10. Rocha V, Labopin M, Sanz G et al. Transplants of umbilical-cord blood or bone marrow from unrelated donors in adults with acute leukemia. N. Engl. J. Med. 2004; 351:2276–85.
CrossRef
11. Eapen M, Rubinstein P, Zhang M-J et al. Outcomes of transplantation of unrelated donor umbilical cord blood and bone marrow in children with acute leukaemia: a comparison study. Lancet 2007; 369:1947–1954.
CrossRef
12. Majhail NS, Weisdorf DJ, Wagner JE et al. Comparable results of umbilical cord blood and HLA-matched sibling donor hematopoietic stem cell transplantation after reduced-intensity preparative regimen for advanced Hodgkin lymphoma. Blood 2006; 107:3804–3807.
CrossRef
13. Burman J, Fransson M, Tötterman TH et al. T-cell responses after haematopoietic stem cell transplantation for aggressive relapsing-remitting multiple sclerosis. Immunology 2013; 140:211–219.
CrossRef
14. Prasad VK, Mendizabal A, Parikh SH et al. Unrelated donor umbilical cord blood transplantation for inherited metabolic disorders in 159 pediatric patients from a single center: influence of cellular composition of the graft on transplantation outcomes. Blood 2008; 112:2979–2989.
CrossRef
15. Bizzetto R, Bonfim C, Rocha V et al. Outcomes after related and unrelated umbilical cord blood transplantation for hereditary bone marrow failure syndromes other than fanconi anemia. Haematologica 2011; 96:134–141.
CrossRef
16. Gluckman E, Anderson V, Ocha R et al. Outcome of cord-blood transplantation from related and unrelated donors. N. Engl. J. Med. 1997; 337:373–381.
CrossRef
17. Ohara Y, Ohto H, Tasaki T et al. Comprehensive technical and patient-care optimization in the management of pediatric apheresis for peripheral blood stem cell harvesting. Transfus. Apher. Sci. 2016; 55: 338-343.
CrossRef
18. Lane BTA, Law P, Maruyama M et al. Harvesting and Enrichment of Hematopoietic Progenitor Cells Mobilized Into the Peripheral Blood. Blood 1995; 85:275–282.
19. Bolan CD, Childs RW, Procter JL et al. Massive immune haemolysis after allogeneic peripheral blood stem cell transplantation with minor ABO incompatibility. Br. J. Haematol. 2001; 112:787–795.
CrossRef
20. Auquier P, Macquart-Moulin G, Moatti JP et al. Comparison of anxiety, pain and discomfort in two procedures of hematopoietic stem cell collection: leukacytapheresis and bone marrow harvest. Bone Marrow Transplant. 1995; 16:541–547.
21. Rossi DJ, Bryder D, Zahn JM et al. Cell Intrinsic Alterations Underlie Hematopoietic Stem Cell Aging. Proc. Natl. Acad. Sci. 2005; 102:9194–9199.
CrossRef
22. Morrison SJ, Wandycz a M, Akashi K et al. The aging of hematopoietic stem cells. Nat.Med. 1996; 2:1011–1016.
CrossRef
23. Ballen KK, Barker JN, Stewart SK et al. Collection and Preservation of Cord Blood for Personal Use. Biol. Blood Marrow Transplant. 2008; 14:356–363.
CrossRef
24. HTA. Guidance document for establishments working with Umbilical cord blood. 2010.
Website
25. Hosing C, Munsell M, Armitage S et al. Ex-Utero Plus in Utero Collection of Umbilical Cord Blood (CB) for Banking Yields Higher Total Nucleated Cell Counts (TNC) Compared to Either Procedure Alone. Biol. Blood Marrow Transplant. 2015; 21:S154–S155.
CrossRef
26. Begley C, Gyte G, Devane D et al. Active versus expectant management for women in the third stage of labour ( Review ). Cochrane Database Syst. Rev. 2015; 9:CD007412.
CrossRef
27. McGuckin CP, Basford C, Hanger K et al. Cord blood revelations-The importance of being a first born girl, big, on time and to a young mother! Early Hum. Dev. 2007; 83:733–741.
CrossRef
28. Nakagawa R, Watanabe T, Kawano Y et al. Analysis of maternal and neonatal factors that influence the nucleated and CD34+ cell yield for cord blood banking. Transfusion 2004; 44:262–267.
CrossRef
29. Surbek D, Visca E, Steinmann C. Umbilical cord blood collection before placental delivery during cesarean delivery increases cord blood volume and nucleated cell number available for transplantation. Am. J. Obstet. Gynecol. 2000; 183:218–221.
CrossRef
30. Askari S, Miller J, Chrysler G et al. Impact of donor- and collection-related variables on product quality in ex utero cord blood banking. Transfusion 2005; 45:189–194.
CrossRef
31. Jones J, Stevens CE, Rubinstein P et al. Obstetric predictors of placental/umbilical cord blood volume for transplantation. Am. J. Obstet. Gynecol. 2003; 188:503–509.
CrossRef
32. Jan RH, Wen SH, Shyr MH et al. Impact of maternal and neonatal factors on CD34+ cell count, total nucleated cells, and volume of cord blood. Pediatr. Transplant. 2008; 12:868–873.
CrossRef
33. Mousavi SH, Abroun S, Zarrabi M et al. The effect of maternal and infant factors on cord blood yield. Pediatr. Blood Cancer 2017; 64:1–8.
CrossRef
34. Manegold G, Meyer-Monard S, Tichelli A et al. Cesarean section due to fetal distress increases the number of stem cells in umbilical cord blood. Transfusion 2008; 48:871–876.
CrossRef
35. Omori A, Takahashi K, Hazawa M et al. Maternal and neonatal factors associated with the high yield of mononuclear low-density/CD34+ cells from placental/umbilical cord blood. Tohoku J. Exp. Med. 2008; 215:23–32.
CrossRef
36. Ballen KK, Klein JP, Pedersen TL et al. Relationship of race/ethnicity and survival after single umbilical cord blood transplantation for adults and children with leukemia and myelodysplastic syndromes. Biol. Blood Marrow Transplant. 2012; 18:903–912.
CrossRef
37. Wen SH, Zhao WL, Lin PY et al. Associations among birth weight, placental weight, gestational period and product quality indicators of umbilical cord blood units. Transfus. Apher. Sci. 2012; 46:39–45.
CrossRef
38. Yang H, Loutfy MR, Mayerhofer S et al. Factors affecting banking quality of umbilical cord blood for transplantation. Transfusion 2011; 51:284–292.
CrossRef
39. Keersmaekers CL, Mason BA, Keersmaekers J et al. Factors affecting umbilical cord blood stem cell suitability for transplantation in an in utero collection program. Transfusion 2014; 54:545–549.
CrossRef
40. Wahid FSA, Nasaruddin MZ, Idris MRM et al. Effects of preeclampsia on the yield of hematopoietic stem cells obtained from umbilical cord blood at delivery. J. Obstet. Gynaecol. Res. 2012; 38:490–497.
CrossRef
41. Kurtzberg J, Wagner EL, Fraser JK et al. Results of the Cord Blood Transplant Study (COBLT) Unrelated Donor Banking Program from Donor Screening to Characterization of Banked Units. Blood 2003; 102:461a.
42. Allan DS, Scrivens N, Lawless T et al. Delayed clamping of the umbilical cord after delivery and implications for public cord blood banking. Transfusion 2016; 56:662–665.
CrossRef
43. Roura S, Pujal J-M, Gálvez-Montón C et al. The role and potential of umbilical cord blood in an era of new therapies: a review. Stem Cell Res. Ther. 2015; 6:123.
CrossRef
44. Clark P, Trickett A, Stark D et al. Factors affecting microbial contamination rate of cord blood collected for transplantation. Transfusion 2012; 52:1770–1777.
CrossRef
45. World Health Organization. Guideline: Delayed umbilical cord clamping for improved maternal and infant health and nutrition outcomes. World Heal. Organ. 2014.
46. Hutton EK, Hassan ES. Late vs early clamping of the umbilical cord in full-term neonates: systematic review and meta-analysis of controlled trials. JAMA 2007; 297:1241–1252.
CrossRef
47. Robertson J. Beyond Survival – 2nd Edition. Pan Am. Heal. Organ. 2013; 73.
48. Yao AC, Lind J, Lu T. Closure of the human umbilical artery: a physiological demonstration of Burton’s theory. Eur. J. Obstet. Gynecol. Reprod. Biol. 1977; 7:365–368.
CrossRef
49. Lapierre V, Pellegrini N, Bardey I et al. Cord blood volume reduction using an automated system (Sepax) vs. a semi-automated system (Optipress II) and a manual method (hydroxyethyl starch sedimentation) for routine cord blood banking: a comparative study. Cytotherapy 2007; 9:165–169.
CrossRef
50. Farid MM, Khudhair AM, Razack SAK et al. A review on phase change energy storage: Materials and applications. Energy Convers. Manag. 2004; 45:1597–1615.
CrossRef
51. Pal R, Hanwate M, Totey SM. Effect of holding time, temperature and different parenteral solutions on viability and functionality of adult bone marrow-derived mesenchymal stem cells before transplantation. J. Tissue Eng. Regen. Med. 2008; 2:436–444.
CrossRef
52. Sousa T, de Sousa ME, Godinho MI et al. Umbilical cord blood processing: volume reduction and recovery of CD34+ cells. Bone Marrow Transpl. 1997; 19:311–313.
CrossRef
53. Armitage S. Cord Blood Processing: Volume Reduction. Cell Preserv. Technol. 2006; 4:9–16.
CrossRef
54. Batista A, Lamontagne D, Chang-Fong S et al. Processing the 5-mL umbilical cord blood unit with the use of an automated wash procedure. Cytotherapy 2015; 17:336–337.
CrossRef
55. Yang H, Acker JP, Abley D et al. High-efficiency volume reduction of cord blood using pentastarch. Bone Marrow Transplant. 2001; 27:457–461.
CrossRef
56. Schwandt S, Korschgen L, Peters S et al. Cord blood collection and processing with hydroxyethyl starch or non-hydroxyethyl starch. Cytotherapy 2016; 18:642–652.
CrossRef
57. Schindler S, Asmus S, von Aulock S et al. Cryopreservation of human whole blood for pyrogenicity testing. J. Immunol. Methods 2004; 294:89–100.
CrossRef
58. Ende M, Ende N. Hematopoietic transplantation by means of fetal (cord) blood. Virginia Med J 1972; 99:276–280.
59. Tse WW, Zang SL, Bunting KD et al. Umbilical cord blood transplantation in adult myeloid leukemia. Bone Marrow Transplant. 2008; 41:465–472.
CrossRef
60. Solh M. Haploidentical vs cord blood transplantation for adults with acute myelogenous leukemia. World J. Stem Cells 2014; 6:371–379.
CrossRef
61. HTA. umbilical cord blood banking A guide for parents. 2016.
62. Rubinstein P, Carrier C, Scaradavou A et al. Outcomes Among 562 Recipients of Placental-Blood Transplants from Unrelated Donors. N. Engl. J. Med 1998; 339:1565–1577.
CrossRef
63. Brunstein CG, Fuchs EJ, Carter SL et al. Alternative donor transplantation after reduced intensity conditioning: Results of parallel phase 2 trials using partially HLA-mismatched related bone marrow or unrelated double umbilical cord blood grafts. Blood 2011; 118:282–288.
CrossRef
64. Labopin M, Ruggeri A, Gorin NC et al. Cost-effectiveness and clinical outcomes of double versus single cord blood transplantation in adults with acute leukemia in France. Haematologica 2014; 99:535–540.
CrossRef
65. Cabral JMS. Ex vivo expansion of hematopoietic stem cells. Biotechnol. Lett. 2001; 23:741–751.
CrossRef
66. United States Food and Drug Administration. Minimal Manipulation of Human Cells, Tissues, and Cellular and Tissue-Based Products. 2014.
67. The European Parliament and the Council of the European Union. Directive 2004/23/EC of the European Parliament and of The Council of 31 March 2004: On setting standards of quality and safety for the donation, procurement, testing, processing, preservation, storage and distribution of human tissues and cells. Off. J. Eur. Union 2004; L 102:
68. Friedestein AJ, Chailakhjan RK, Lalykina KS. The development of fibroblast colonies in monolayer cultures of guinea-pig bone marrow and spleen cells. Cell Tissue Kinet. 1970; 3:393–403.
69. Baiguera S, Jungebluth P, Mazzanti B et al. Mesenchymal stromal cells for tissue-engineered tissue and organ replacements. Transpl. Int. 2012; 25:369–382.
CrossRef
70. da Silva Meirelles L, Chagastelles PC, Nardi NB. Mesenchymal stem cells reside in virtually all post-natal organs and tissues. J. Cell Sci. 2006; 119:2204–13.
CrossRef
71. Thirumala S, Goebel WS, Woods EJ. Manufacturing and banking of mesenchymal stem cells. Expert Opin. Biol. Ther. 2013; 13:673–91.
CrossRef
72. Gornostaeva A, Andreeva E, Buravkova L. Factors governing the immunosuppressive effects of multipotent mesenchymal stromal cells in vitro. Cytotechnology 2015; 68:1515–1527.
73. Fazzina R, Mariotti A, Procoli A et al. A new standardized clinical-grade protocol for banking human umbilical cord tissue cells. Transfusion 2015; 55:2864–2873.
CrossRef
74. Iftimia-Mander A, Hourd P, Dainty R et al. Mesenchymal stem cell isolation from human umbilical cord tissue: understanding and minimizing variability in cell yield for process optimization. Biopreserv. Biobank. 2013; 11:291–8.
CrossRef
75. Stewart PS, Costerton JW. Antibiotic resistance of bacteria in biofilms. Lancet 2001; 358:135–138.
CrossRef
76. Lim J, Razi ZRM, Law J et al. MSCs can be differentially isolated from maternal, middle and fetal segments of the human umbilical cord. Cytotherapy 2016; 18:1493–1502.
CrossRef
77. Kim J, Piao Y, Pak YK et al. Umbilical cord mesenchymal stromal cells affected by gestational diabetes mellitus display premature aging and mitochondrial dysfunction. Stem Cells Dev. 2015; 24:575–586.
CrossRef
78. Huang S, Feng C, Wu Y et al. Dissimilar characteristics of umbilical cord mesenchymal stem cells from donors of different ages. Cell Tissue Bank. 2013; 14:707–713.
CrossRef
79. Pichichero ME, Pichichero DM. Diagnosis of penicillin, amoxicillin, and cephalosporin allergy: Reliability of examination assessed by skin testing and oral challenge. J. Pediatr. 1998; 132:137–143.
CrossRef
80. Hak AM, Offerijns FGJ, Verheul CC. Toxic effects of DMSO on cultured beating heart cells at temperatures above zero. Cryobiology 1973; 10:244–250.
CrossRef
81. Rowley SD, Feng Z, Yadock D et al. Post-thaw removal of DMSO does not completely abrogate infusional toxicity or the need for pre-infusion histamine blockade. Cytotherapy 1999; 1:439–446.
CrossRef
82. Galvao J, Davis B, Tilley M et al. Unexpected low-dose toxicity of the universal solvent DMSO. FASEB J. 2014; 28:1317–1330.
CrossRef
83. Jung S, Panchalingam KM, Rosenberg L et al. Ex vivo expansion of human mesenchymal stem cells in defined serum-free media. Stem Cells Int. 2012; 2012: 123030.
CrossRef
84. Panchalingam KM, Jung S, Rosenberg L et al. Bioprocessing strategies for the large-scale production of human mesenchymal stem cells: a review. Stem Cell Res. Ther. 2015; 6:225.
CrossRef
85. Torre ML, Lucarelli E, Guidi S et al. Ex Vivo Expanded Mesenchymal Stromal Cell Minimal Quality Requirements for Clinical Application. Stem Cells Dev. 2015; 24:677–685.
CrossRef
86. Dong-rui L, Jian-hui C. Methods of isolation, expansion, differentiating induction and preservation of human umbilical cord mesenchymal stem cells. Chin. Med. J. (Engl). 2012; 125:4504–4510.
87. de Soure AM, Fernandes-Platzgummer A, Moreira F et al. Integrated culture platform based on a human platelet lysate supplement for the isolation and scalable manufacturing of umbilical cord matrix-derived mesenchymal stem/stromal cells. J. Tissue Eng. Regen. Med. 2017; 11: 1630-1640.
CrossRef
88. La Rocca G, Anzalone R, Corrao S et al. Isolation and characterization of Oct-4+/HLA-G+ mesenchymal stem cells from human umbilical cord matrix: Differentiation potential and detection of new markers. Histochem. Cell Biol. 2009; 131:267–282.
CrossRef
89. Lu L, Liu Y, Yang S et al. Isolation and characterization of human umbilical cord mesenchymal stem cells with hematopoiesis-supportive function and other potentials. Haematologica 2006; 91:1017–1026.
90. Zhang H, Zhang B, Tao Y et al. Isolation and characterization of mesenchymal stem cells from whole human umbilical cord applying a single enzyme approach. Cell Biochem. Funct. 2012; 30:643–649.
CrossRef
91. Han Y-F, Tao R, Sun T-J et al. Optimization of human umbilical cord mesenchymal stem cell isolation and culture methods. Cytotechnology 2013; 65:819–27.
CrossRef
92. Mori Y, Ohshimo J, Shimazu T et al. Improved Explant Method To Isolate Umbilical Cord-derived Mesenchymal Stem Cells And Their Immunosuppressive Properties. Tissue Eng. Part C. Methods 2014; 21:5–9.
93. Romanov YA, Balashova EE, Volgina NE et al. Optimized Protocol for Isolation of Multipotent Mesenchymal Stromal Cells from Human Umbilical Cord. Bull. Exp. Biol. Med. 2015; 160:148–154.
CrossRef
94. Davies JE, Walker JT, Keating A. Concise Review: Wharton’s Jelly: The Rich, but Enigmatic, Source of Mesenchymal Stromal Cells. Stem Cells Transl. Med. 2017; 6:1620-1630.
CrossRef
95. Patel AN, Vargas V, Revello P et al. Mesenchymal stem cell population isolated from the subepithelial layer of umbilical cord tissue. Cell Transplant. 2013; 22:513–519.
CrossRef
96. Davies JE, Baksh D, Sarugaser R et al. WO2004/072273: Progenitor cells from Wharton’s jelly of human umbilical cord. 2004;
97. Pereira T, Silva PASA, Amorim I et al. Effects of Human Mesenchymal Stem Cells Isolated from Wharton’s Jelly of the Umbilical Cord and Conditioned Media on Skeletal Muscle Regeneration Using a Myectomy Model. Stem Cells Int. 2014; 376918.
CrossRef
98. FDA. Considerations for the Design of Early-Phase Clinical Trials of Cellular and Gene Therapy Products. 2015; 1–24.
99. European Commission D. Annex. EU Guidelines for Good Manufacturing Practice for Medicinal Products for Human and Veterinary Use. 2015; 4:1–16.
100. Use V. EU GMP Guideline, Volume 4, Chapter 3: Premises and Equipment. 2016; 1–5.
101. Adams D, Quayum M, Worthington T et al. Evaluation of a 2% chlorhexidine gluconate in 70% isopropyl alcohol skin disinfectant. J. Hosp. Infect. 2005; 61:287–290.
CrossRef
102. Gardini F, Martuscelli M, Caruso MC et al. Effects of pH, temperature and NaCl concentration on the growth kinetics, proteolytic activity and biogenic amine production of Enterococcus faecalis. Int. J. Food Microbiol. 2001; 64:105–117.
CrossRef
103. Martínez S, López M, Bernardo A. Thermal inactivation of Enterococcus faecium: Effect of growth temperature and physiological state of microbial cells. Lett. Appl. Microbiol. 2003; 37:475–481.
CrossRef
104. Da-Croce L, Gambarini-Paiva GHR, Angelo PC et al. Comparison of vitrification and slow cooling for umbilical tissues. Cell Tissue Bank. 2013; 14:65–76.
CrossRef
105. Fry LJ, Querol S, Gomez SG et al. Assessing the toxic effects of DMSO on cord blood to determine exposure time limits and the optimum concentration for cryopreservation. Vox Sang. 2015; 109:181–190.
CrossRef
106. Halle P, Tournilhac O, Knopinska-Posluszny W et al. Uncontrolled-rate freezing and storage at-80°C, with only 3.5-percent DMSO in cryoprotective solution for 109 autologous peripheral blood progenitor cell transplantations. Transfusion 2001; 41:667–673.
CrossRef
107. Rodríguez L, Velasco B, García J et al. Evaluation of an automated cell processing device to reduce the dimethyl sulfoxide from hematopoietic grafts after thawing. Transfusion 2005; 45:1391–1397.
CrossRef
108. Motta JPR, Gomes BE, Bouzas LF et al. Evaluations of bioantioxidants in cryopreservation of umbilical cord blood using natural cryoprotectants and low concentrations of dimethylsulfoxide. Cryobiology 2010; 60:301–307.
CrossRef
109. Schwandt S, Korschgen L, Peters S et al. Cord blood collection and processing with hydroxyethyl starch or non-hydroxyethyl starch. Cytotherapy 2016; 18:642–652.
CrossRef
110. Chen G, Yue A, Ruan Z et al. Comparison of the Effects of Different Cryoprotectants on Stem Cells from Umbilical Cord Blood. Stem Cells Int. 2016; 1–7.
CrossRef
111. Marquez-Curtis LA, Janowska-Wieczorek A, McGann LE et al. Mesenchymal stromal cells derived from various tissues: Biological, clinical and cryopreservation aspects. Cryobiology 2015; 71:181–197.
CrossRef
112. Kusuma I, Hadi RS, Kiranadi B et al. Trehalose preincubation increases mesenchymal (CD271+) stem cells post-cryopreservation viability. Med. J. Indones. 2016; 25:129–135.
CrossRef
113. Sensebé L, Gadelorge M, Fleury-Cappellesso S. Production of mesenchymal stromal/stem cells according to good manufacturing practices: a review. Stem Cell Res. Ther. 2013; 4:66.
CrossRef
114. Swamynathan P, Venugopal P, Kannan S et al. Are serum-free and xeno-free culture conditions ideal for large scale clinical grade expansion of Wharton’s jelly derived mesenchymal stem cells? A comparative study. Stem Cell Res. Ther. 2014; 5:88.
CrossRef
115. Booth GS, Gehrie EA, Bolan CD et al. Clinical Guide to ABO-Incompatible Allogeneic Stem Cell Transplantation. Biol. Blood Marrow Transplant. 2013; 19:1152–1158.
CrossRef
116. Heathman TRJ, Nienow AW, McCall MJ et al. The Translation of Cell-Based Therapies: Clinical Landscape and Manufacturing Challenges. Regen. Med. 2015; 10:49–64.
CrossRef
117. Sideri A, Neokleous N, Grange PB de la G et al. An overview of the progress on double umbilical cord blood transplantation. Haematologica 2011; 96:1213–1220.
CrossRef
118. Cutler C, Ballen K. Reduced-intensity conditioning and umbilical cord blood transplantation in adults. Bone Marrow Transplant. 2009; 44:667–671.
CrossRef
119. Parikh SH, Mendizabal A, Benjamin CL et al. A novel reduced-intensity conditioning regimen for unrelated umbilical cord blood transplantation in children with nonmalignant diseases. Biol. Blood Marrow Transplant. 2014; 20:326–336.
CrossRef
120. Okada M, Yoshihara S, Taniguchi K et al. Intrabone marrow transplantation of unwashed cord blood using reduced-intensity conditioning treatment: A Phase I study. Biol. Blood Marrow Transplant. 2012; 18:633–639.
CrossRef
121. Frassoni F, Gualandi F, Podest M et al. Direct intrabone transplant of unrelated cord-blood cells in acute leukaemia: a phase I/II study. Lancet Oncol. 2008; 9:831–839.
CrossRef
122. Macmillan ML, Blazar BR, Defor TE et al. Transplantation of ex-vivo culture-expanded parental haploidentical mesenchymal stem cells to promote engraftment in pediatric recipients of unrelated donor umbilical cord blood: results of a phase I-II clinical trial. Bone Marrow Transplant. 2009; 43:447–454.
CrossRef
123. Battiwalla M, Hematti P. Mesenchymal Stem Cells in Hematopoietic Stem Cell Transplantation. Cryotherapy 2010; 11:503–515.
CrossRef
124. Dominici M, Le Blanc K, Mueller I et al. Minimal criteria for defining multipotent mesenchymal stromal cells. The International Society for Cellular Therapy position statement. Cytotherapy 2006; 8:315–7.
CrossRef
Affiliations
Lindsey Parker1,2, Shaun Mansfield2, Kate Sneddon2, Ben Charles2 & Qasim A Rafiq*1
1 Advanced Centre for Biochemical Engineering, Department of Biochemical Engineering, University College London, Gower Street, London, WC1E 6BT, UK
2 Biovault Technical Ltd, Plymouth International Medical & Technology Park, 24 Brest Road, Derriford, Plymouth, PL6 5XP, UK
*Author for Correspondence:
q.rafiq@ucl.ac.uk
This work is licensed under a Creative Commons Attribution- NonCommercial – NoDerivatives 4.0 International License.