Mouse mammary tumor virus-based vector for efficient & safe transgene delivery into mitotic & non-mitotic cells
Cell Gene Therapy Insights 2016; 2(5), 589-598.
10.18609/cgti.2016.062
Retroviruses are cellular parasites that have evolved to deliver and integrate their nucleic acids to the host chromosomes. Therefore, they have been converted into gene delivery vehicles (vectors) and used for the effective transduction of genes in tissue culture cells as well, as for therapeutic gene transfer approaches. Until recently, vectors derived from mouse mammary tumor virus (MMTV) were inefficient in gene delivery. However, recent advances in betaretrovirology revealed the underlying obstacles that prevented the conversion of MMTV to an efficient gene delivery vehicle and allowed the construction of a high-titer MMTV-based vector system with a split genome design. The vector combines several desirable properties of retroviral vectors such as random integration into the host chromosomes and the capability to transduce genes into non-dividing cells. This article will discuss the latest developments and the remaining challenges in utilizing MMTV-based vectors in gene delivery.
Retrovirus-based vector development
The field of retroviral vector development is evolving rapidly. Since the introduction of the first retrovirus-based vectors in the early 1980s, they have been widely used for gene or protein delivery into cells in vitro as well as in vivo [1–3]. Due to the unique characteristics of retroviruses, retrovirus-based means of gene or protein delivery are superior (with respect to efficiency) to non-viral transfer methods. Detailed knowledge of retrovirus biology, including an understanding of the retrovirus life cycle, genome structure and virus particle organization, paved the way for the development of various retrovirus-based technologies for the delivery of genes or proteins to target cells. This includes the generation of: integration-competent retrovirus vectors; integration incompetent episome transfer vehicles; mRNA delivery tools; and finally protein transfer vector particles. The different technologies can also be combined to achieve co-delivery of a protein of interest, together with an RNA or a donor DNA template.
Retrovirus-based vectors have been developed from members of all seven genera of the Retroviridae family. The most commonly used vectors in the laboratory and clinical practice were developed from gammaretroviruses (mostly derived from murine leukemia virus [MLV]) and lentiviruses (mostly derived from human immunodeficiency virus type-1 [HIV-1]). They are, in most cases, used for long-term transgene expression and permanent cell modification. Historically, gammaretroviral vectors were the first vectors to be used in gene therapy clinical trials. Due to a simple genome organization, knowledge of the complete MLV sequence and the establishment of a stable packaging cell line for the production of replication-incompetent retroviral particles, MLV-based vectors were used to correct genetic defects of immunocompromised patients [4–6]. Stably corrected immunological phenotype in the vast majority of the patients confirmed applicability of retrovirus-based gene delivery for gene therapy applications. However, these clinical trials also uncovered drawbacks of the early generation MLV-based vectors; namely, their potential genotoxicity leading to adverse effects that culminated in tumorigenesis in some human patients [7]. Since then, the safety profile of MLV-based vectors has been markedly improved. The long terminal repeats (LTRs) have been modified such that after vector integration two promoter- and enhancer-less LTRs flank the transgene of interest whose expression is driven by a heterologous promoter [8]. Furthermore, insulator sequences have been inserted into the residual U3 regions of the 3´LTR to further reduce any undesired effects of insertional mutagenesis. Great effort has been invested into the characterization of the undesired propensity of the gammaretroviral vectors to integrate in the vicinity of the promoter and enhancer regions in the host chromosomes. Cellular BET proteins were found to interact with MLV integrase and direct the virus integration into the transcription start sites [9–11]. Understanding of the underlying mechanism responsible for the non-random promoter-directed MLV integration profile may pave a way to re-directing integration to safer genomic loci. Another limitation of gammaretroviral vectors is their inability to transduce genetic material to non-dividing cells. This stems from the inability of the MLV pre-integration complex (PIC) to traverse the nuclear pores [12]. Thus, breakdown of the nuclear membrane during mitosis is essential for entry of the MLV DNA into the host genomes. Most tissues consist of differentiated, non-dividing cells, therefore MLV-based vectors are not applicable for gene delivery to these tissues.
This restriction does not apply to vectors derived from lentiviruses. They can cross the intact nuclear membrane via the nuclear pore complexes and access the host cell chromatin of non-mitotic cells. The ability of lentiviral vectors to transduce non-dividing cells is given by the fact that HIV-1 and other lentiviruses can replicate in certain quiescent or terminally differentiated cells such as macrophages or microglia [13,14]. The lentiviral PICs carry karyophilic signals mediating interaction with the components of cellular nuclear import machinery such as TNPO3 and RANBP2 proteins leading to translocation of PICs across the nuclear membrane [15,16]. Due to this property, lentivirus-based vector have been developed to transduce genes into hard-to-transduce cells including neurons and hematopoietic stem cells [17,18], and have thus become the most popular vectors for use in retrovirus-based gene therapy trials. Apart from their ability to transduce cells regardless of cell cycle status, lentiviral vectors are also commonly used due to their integration pattern, which is distinct from that of gammaretroviruses. Specifically, lentiviruses do not preferentially integrate near enhancers, transcription start sites, CpG islands and DNase I hypersensitive regions. Instead, their integration is targeted to the body of active transcription units [19,20]. Up to 80% of the newly integrated proviruses can be found in genes. This targeting is achieved through interaction of the HIV-1 integrase with a transcriptional coactivator – lens epithelium-derived growth factor (LEDGF/p75) [21–23]. Although the integration within genes is considered to be less genotoxic than integration directed to promoter/enhancer regions, it still represents a considerable risk of causing malignant transformations. Thus, vectors exhibiting a neutral integration pattern may represent an alternative to current commonly used platforms. One of the recently developed vectors integrating randomly into the host genome is a vector derived from avian sarcoma-leukosis virus (ASLV) – alpharetroviral vector (AVV) [19,24]. Another recently developed vector with a random integration profile is a vector derived from the prototypic betaretrovirus, mouse mammary tumor virus (MMTV) [25, 26].
Mouse mammary tumor virus biology
MMTV was discovered in the late 1930s as a virus transmitted from infected mothers via milk to newborn mice [27]. During milk-borne infection, the virus first infects dendritic cells (DCs) in the small intestine and Peyer’s patches and then spreads to T and B cells [28]. A MMTV accessory protein, superantigen (Sag), is presented by the MHCII proteins on the surface of the infected B cells and DCs to T cells. The Sag-activated T cells divide, secrete cytokines that stimulate the infected B cells for proliferation and recruit additional T and B cells to the lymphoid compartment. The amplification of pools of the infected lymphocytes is critical for the virus to spread to its ultimate target – the mammary gland [29,30].
The genome of MMTV, much like the genome of other retroviruses, encodes the structural components of virions (matrix/capsid/nucleocapsid), the enzymes required for virus replication (reverse transcriptase/integrase) and the envelope protein responsible for virus binding to the cell surface and its entry (Figure 1). Additionally, MMTV encodes at least three accessory non-structural proteins that facilitate virus replication in the host. The MMTV genome is 9 kb in length and like all retroviruses is flanked by the 5´- and 3´ LTRs. The LTRs are exceptionally long (1.4 kb) because they contain an open reading frame encoding the viral accessory protein Sag. In addition, the LTR carries a number of transcription factor binding sites that determine tissue-specific and hormone-dependent (glucocorticoid; progesterone) virus gene expression.
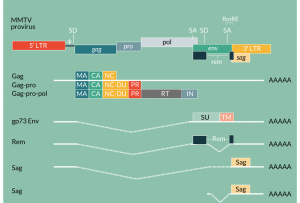
Schematic representation of the MMTV proviral genome and the MMTV RNAs. The full length RNA (9 kb) serves as the virus genome and mRNA. It contains a 5´cap and a 3´poly-A tail and is can be translated to Gag, Gag-Pro and Gag-Pro-Pol polyproteins that are processed by the virus protease (PR) to matrix (MA), capsid (CA), nucleocapsid (NC), nucleocapsid-dUTPase (NC-DU), reverse transcriptase (RT) and integrase (IN) proteins. The singly spliced mRNA encodes gp73/Env proteins that are processed by furin protease to surface (SU, gp52) and transmembrane (TM, gp36) subunits. The doubly spliced mRNA encodes the regulator of MMTV expression, Rem that binds to RmRE and facilitates nuclear export of the unspliced RNA. The superantigen protein (Sag) is encoded by the 3´end of the genome.
At least five transcripts are generated from the MMTV genome. A full-length, unspliced RNA serves either as the virus genome that is packaged into viral particles or as the transcript translated into Gag, Gag-Pro and Gag-Pro-Pol polyproteins (Figure 1). The production of the latter two polyproteins is dependent on a frameshift suppression during which the ribosome must back up by one base to continue translation in the alternate frame. The efficiency of frameshifting seems to be higher for MMTV RNA than for RNAs from other retroviruses (e.g., ASLV), because the equivalent amount of the Gag-Pro-Pol precursor is synthesized even though two frameshift events are required [31].
As with other complex retroviruses, MMTV encodes several accessory proteins that affect the immune system of the host (e.g., Sag protein) and facilitates replication of MMTV in cells (Figure 1). A deoxyuridine-triphosphatese (dUTPase [DU]) is encoded by the full-length transcript and translated in the pro frame. The enzyme, which catalyzes hydrolysis of dUTP to dUMP and diphosphate, is produced following proteolytic cleavage of the Gag-Pro and Gag-Pro-Pol polyproteins. The dUTPases remove dUTPs from the deoxynucleotide (dNTP) pool thereby reducing the probability of incorporation of this base into DNA during reverse transcription. It is believed that the role of the enzyme is especially important in cells with a low concentration of dNTPs such as non-dividing cells (macrophages, DCs).
MMTV also encodes an accessory protein analogous to the HIV-1 Rev: Rem. Rem is encoded by doubly spliced mRNA and, like Rev, facilitates the transport of unspliced viral transcripts containing the Rem responsive element (RmRE) from the nucleus to the cytoplasm. Thus, Rem is a crucial regulator of the expression of viral genes.
MMTV entry into cells is mediated by the Env protein encoded by a singly spliced mRNA. The synthesized Env (gp73) polyprotein precursor is cleaved by cellular furin protease into surface (SU, gp52) and transmembrane (TM, gp36) subunits. The gp52 binds to the mouse transferrin receptor 1 (TfR1) that is then used as a virus entry receptor [32]. TfR1 is a ubiquitously expressed carrier protein used for the import of iron into the cell. Importantly, entry of MMTV to cells, mediated by gp52-TfR1 interaction, is rather inefficient [32,33]. Thus, the usage of the MMTV Env in virus vectors is limited.
Development of MMTV-based vector systems
The above described attributes led to the development of the third generation of MMTV-based vectors. It differs from the early generations in that it carries a number of safety features. Additionally, the third-generation vectors can be produced at markedly higher titers comparable to lentiviral vectors.
Historically, the first described MMTV vectors were derived from a complete molecular clone. They were replication deficient and carried a marker expression cassette either in the Pol/Env coding regions or in the 3´LTR. The vector particles were produced by transfecting the vector construct into MMTV-infected helper cells or, in case of the complete molecular clone carrying the transgene in the 3´LTR, into cells lacking MMTV sequences [34–37]. A split-genome design (second generation) was for the first time used by Salmons and colleagues who developed a packaging cell line expressing Gag-Pro-Pol and Env from a heterologous RSV promoter [38]. Further improvement of the split genome system was achieved by introducing a hybrid CMV-R/U5 promoter into the vector construct to completely overcome the need for hormonal stimulation that is required for the RNA synthesis from the authentic MMTV promoter [39]. In the early generation vectors, the authentic MMTV Env protein was used to mediate virus entry. However, as mentioned above, the MMTV Env-mediated vector entry resulted in low virus titers in murine as well as non-murine target cells. The titer did not markedly improve when an MMTV Env mutant, optimized for entry to human cells, was used [33]. Thus, the development of a high-titer vector necessitated the usage of pseudotyped vector particles [Unpublished Data]. Another drawback of the first- and second-generation vectors was insufficient transport of viral RNAs to the cytoplasm. The viral proteins were not produced at high levels resulting in a low infectivity of the MMTV vectors (titer ≤103 transduction units [TU]/ml) [35]. This resulted from the fact that MMTV was considered to be a simple retrovirus analogous to MLV. Discovery of Rem and its function opened up new possibilities for vector improvements [40–43].
High-titer vector preparations (>105 TU/ml) were obtained by developing the third-generation MMTV vector system. The vector genome and helper functions (Gag-Pro-Pol, Env, Rem or Rev) were expressed from four separate plasmids containing a strong constitutive promoter (CMV or RSV) (Figure 2). To produce vector transcripts, the U3 region of the 5´LTR was replaced with the RSV promoter such that transcription initiates at the authentic transcription start site. This modification resulted in a vector where high-level transcription is independent of the presence of glucocorticoid hormones. To further improve vector efficiency and safety, the cis-acting sequences required for efficient gene transfer were mapped and nonessential regions were removed. This included the enhancer region in the 3´LTR, resulting in a self-inactivating (SIN) vector lacking the sag gene. Special attention was paid to preserving signals involved in the regulation of mRNA polyadenylation, such as CPSF, CstF, CF Im and U-rich region, as it is generally known that some SIN vectors suffer from leaky transcriptional termination [44,45]. To stabilize vector RNA and facilitate export from the nucleus, two post-transcriptional regulatory elements were inserted into the vector. The woodchuck hepatitis virus post-transcriptional regulatory element was inserted upstream of the 3´LTR and the Rev responsive element (RRE) was placed downstream of the packaging signal (ψ) that has been mapped to a region spanning the 5´UTR/gag junction [46,47].
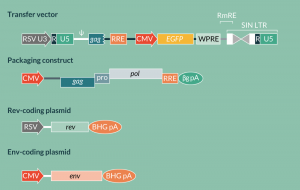
Schematic representation of the four plasmid expression system for generation of a pseudotyped MMTV-based vector by transient transfection of HEK293T cells. A constitutive promoter (CMV or RSV) drives the expression of vector RNA and helper proteins. A large portion of the 3´LTR has been deleted resulting in a self-inactivating (SIN) vector that loses the capacity to drive the expression of vector RNA following reverse transcription. The expression of transgene (EGFP) is driven by an internal constitutive promoter. Stability of the vector RNA is enhanced by the woodchuck hepatitis post-transcriptional element (WPRE) and the nuclear export is facilitated in the presence of Rev by a cis-acting element – Rev-responsive element (RRE). The expression of the structural and enzymatic components of the MMTV vector is also facilitated by the Rev-RRE interaction. The Rev and Env (VSV-G) proteins are expressed in trans.
As mentioned above, the MMTV Env is not efficient in mediating vector entry. Therefore, a heterologous envelope was used to enhance vector infectivity. The vesicular stomatitis virus glycoprotein (VSV-G), previously shown to support the infectivity of MMTV cores, was employed to pseudotype vector particles [39]. The pseudotyping combined with the implementation of post-transcriptional regulatory functions resulted in a marked increase of vector titers relative to the previous generations (100–1000-fold increase; titer >105 TU/ml). Additionally, the pseudoparticles were stable and they could be efficiently concentrated by ultracentrifugation leading to further increase in vector titers (titer >107 TU/ml) [26].
A high-vector titer is essential for clinically relevant applications as well as to gain deeper insight into the virus biology. We used the third-generation vector to investigate whether MMTV, like HIV-1, is capable of infecting non-dividing cells. In vivo, MMTV infects terminally differentiated dendritic cells and uses dUTPase to avoid misincorporation of dUTPs into the nascent DNA during reverse transcription. This evidence suggests that MMTV infects non-mitotic cells. We used cell cycle-arrested tissue culture cells (a high dose of γ-irradiation) and unstimulated hematopoietic stem cells (human CD34+, mouse Lin-) to test if the virus can infect cells regardless of cell cycle progression. Importantly, the MMTV vector transduced the eGFP gene into the non-dividing cells with the same efficiency as the control HIV-1 vector. Thus, we concluded that MMTV infects non-dividing cells [26].
However, in contrast to HIV-1 vector, monitoring of integration sites showed that the vector derived from the prototypic betaretrovirus has a more favorable integration pattern. Specifically, the vector exhibited in dividing and non-dividing cells a close-to-random integration site distribution [26]. This result confirmed previously observed unbiased integration profile for the wild-type virus [25,48]. Thus, in contrast to lentivirus- and gammaretrovirus-derived vectors, most of the integrations occurred in non-coding regions of the genome. The underlying mechanism responsible for this integration pattern is currently unknown. MMTV seems to use a different nucleus entry pathway than HIV-1. This pathway is independent of a nuclear import factor for serine/arginine-rich proteins (TNPO3) that was shown to interact with HIV-1 CA and mediate the HIV-1 nuclear import [26,49,50]. Moreover, MMTV does not interact with another cellular factor that directs localization of the HIV-1 PICs in the host nucleus, LEDGF/p75 [Data not shown]. Work to identify a cellular factor that tethers the MMTV PICs to the host chromatin is currently ongoing, as characterization of this chromatin tethering mechanism may be beneficial for the improvement of the integration profile, and thus may lead to targeted integration.
Translational insight
The unique properties of MMTV have been exploited for the development of a new platform for efficient gene delivery to target cells. The vector combines several safety features such as: split genome design that minimizes the possibility of reconstitution of a replication-competent virus; deletion of a large portion of the 3´LTR, resulting in a SIN vector that has a low potential to transactivate nearby genes and does not contain sag gene; and the random integration profile that decreases the chance of unwanted integration into genes that is commonly observed with other vectors.
Additionally, MMTV vector does not require mitosis for efficient transduction. This property makes the vector system superior to many other vectors that require mitosis and their therapeutic application is thus limited to mitotic cells. The ability of MMTV to transduce long-term repopulating cells has been demonstrated, providing the basis for ex vivo applications such as hematopoietic stem cells gene therapy. To date, no in vivo experiments have been performed with the new MMTV vector system. These trials are needed to demonstrate lower genotoxicity of the vector compared to other vectors. Such studies will hopefully establish MMTV vector as an efficient and safe alternative to gammaretroviral and lentivirial vector systems.
Financial & competing interests disclosure
The author has no relevant financial involvement with an organization or entity with a financial interest in or financial conflict with the subject matter or materials discussed in the manuscript. This includes employment, consultancies, honoraria, stock options or ownership, expert testimony, grants or patents received or pending, or royalties. No writing assistance was utilized in the production of this manuscript.
References
1. Mann R, Mulligan RC, Baltimore D. Construction of a retrovirus packaging mutant and its use to produce helper-free defective retrovirus. Cell 1983; 33: 153–9.
CrossRef
2. Watanabe S, Temin HM. Construction of a helper cell line for avian reticuloendotheliosis virus cloning vectors. Mol. Cell Biol. 1983; 3: 2241–9.
CrossRef
3. Wei CM, Gibson M, Spear PG, Scolnick EM. Construction and isolation of a transmissible retrovirus containing the src gene of Harvey murine sarcoma virus and the thymidine kinase gene of herpes simplex virus type 1. J. Virol. 1981; 39: 935–44.
Website
4. Cavazzana-Calvo M, Hacein-Bey S, de Saint Basile G et al. Gene therapy of human severe combined immunodeficiency (SCID)-X1 disease. Science 2000; 288: 669–672.
CrossRef
5. Chinen J, Davis J, De Ravin SS et al. Gene therapy improves immune function in preadolescents with X-linked severe combined immunodeficiency. Blood 2007; 110: 67–73.
CrossRef
6. Gaspar HB, Parsley KL, Howe S et al. Gene therapy of X-linked severe combined immunodeficiency by use of a pseudotyped gammaretroviral vector. Lancet 2004; 364: 2181–7.
CrossRef
7. Hacein-Bey-Abina S, von Kalle C, Schmidt M et al. A serious adverse event after successful gene therapy for X-linked severe combined immunodeficiency. N. Engl. J. Med. 2003; 348: 255–6.
CrossRef
8. Yu SF, von Ruden T, Kantoff PW et al. Self-inactivating retroviral vectors designed for transfer of whole genes into mammalian cells. Proc. Natl Acad. Sci. USA 1986; 83: 3194–8.
CrossRef
9. De Rijck J, de Kogel C, Demeulemeester J et al. The BET family of proteins targets moloney murine leukemia virus integration near transcription start sites. Cell Rep. 2013; 5: 886–94.
CrossRef
10. Gupta SS, Maetzig T, Maertens GN et al. Bromo- and extraterminal domain chromatin regulators serve as cofactors for murine leukemia virus integration. J. Virol. 2013; 87: 12721–36.
CrossRef
11. Sharma A, Larue RC, Plumb MR et al. BET proteins promote efficient murine leukemia virus integration at transcription start sites. Proc. Natl Acad. Sci. USA 2013; 110: 12036–41.
CrossRef
12. Miller DG, Adam MA, Miller AD. Gene transfer by retrovirus vectors occurs only in cells that are actively replicating at the time of infection. Mol. Cell Biol. 1990; 10: 4239–42.
CrossRef
13. Lewis P, Hensel M, Emerman M. Human immunodeficiency virus infection of cells arrested in the cell cycle. EMBO J. 1992; 11: 3053–8.
Website
14. Weinberg JB, Matthews TJ, Cullen BR, Malim MH. Productive human immunodeficiency virus type 1 (HIV-1) infection of nonproliferating human monocytes. J. Exp. Med. 1991; 174: 1477–82.
CrossRef
15. Bukrinsky MI, Sharova N, Dempsey MP et al. Active nuclear import of human immunodeficiency virus type 1 preintegration complexes. Proc. Natl Acad. Sci. USA 1992; 89: 6580–4.
CrossRef
16. Bukrinsky MI, Haggerty S, Dempsey MP et al. A nuclear localization signal within HIV-1 matrix protein that governs infection of non-dividing cells. Nature 1993; 365: 666–9.
CrossRef
17. Naldini L, Blomer U, Gage FH, Trono D, Verma IM. Efficient transfer, integration, and sustained long-term expression of the transgene in adult rat brains injected with a lentiviral vector. Proc. Natl Acad. Sci. USA 1996; 93: 11382–8.
CrossRef
18. Naldini L, Blomer U, Gallay P et al. In vivo gene delivery and stable transduction of nondividing cells by a lentiviral vector. Science 1996; 272: 263–7.
CrossRef
19. Mitchell RS, Beitzel BF, Schroder AR et al. Retroviral DNA Integration, ASLV, HIV, and MLV Show Distinct Target Site Preferences. PLoS Biol. 2004; 2: E234.
CrossRef
20. Wu X, Li Y, Crise B, Burgess SM. Transcription start regions in the human genome are favored targets for MLV integration. Science 2003; 300: 1749–51.
CrossRef
21. Cherepanov P, Maertens G, Proost P et al. HIV-1 integrase forms stable tetramers and associates with LEDGF/p75 protein in human cells. J. Biol. Chem. 2003; 278: 372–81.
CrossRef
22. Ciuffi A, Llano M, Poeschla E et al. A role for LEDGF/p75 in targeting HIV DNA integration. Nat. Med. 2005; 11, 1287–9.
CrossRef
23. Llano M, Saenz DT, Meehan A et al. An essential role for LEDGF/p75 in HIV integration. Science 2006; 314: 461–4.
CrossRef
24. Suerth JD, Maetzig T, Galla M, Baum C, Schambach A. Self-inactivating alpharetroviral vectors with a split-packaging design. J. Virol. 2010; 84: 6626–35.
CrossRef
25. Faschinger A, Rouault F, Sollner J et al. Mouse mammary tumor virus integration site selection in human and mouse genomes. J. Virol. 2008; 82: 1360–7.
CrossRef
26. Konstantoulas CJ, Indik S. Mouse mammary tumor virus-based vector transduces non-dividing cells, enters the nucleus via a TNPO3-independent pathway and integrates in a less biased fashion than other retroviruses. Retrovirology 2014; 11: 34.
CrossRef
27. Bittner JJ. Some possible effects of nursing on the mammary gland tumor incidence in mice. Science 1936; 84: 162.
CrossRef
28. Burzyn D, Rassa JC, Kim D et al. Toll-like receptor 4-dependent activation of dendritic cells by a retrovirus. J. Virol. 2004; 78: 576–84.
CrossRef
29. Finke D, Acha-Orbea H. Differential migration of in vivo primed B and T lymphocytes to lymphoid and non-lymphoid organs. Eur. J. Immunol. 2001; 31: 2603–11.
30. Golovkina TV, Dudley JP, Ross SR. B and T cells are required for mouse mammary tumor virus spread within the mammary gland. J. Immunol. 1998; 161: 2375–82.
Website
31. Coffin JM, Hughes SH, Varmus HE. Retroviruses. Cold Spring Harbor Laboratory Press (1997).
32. Ross SR, Schofield JJ, Farr CJ, Bucan M. Mouse transferrin receptor 1 is the cell entry receptor for mouse mammary tumor virus. Proc. Natl Acad. Sci. USA 2002; 99: 12386–90.
CrossRef
33. Konstantoulas CJ, Lamp B, Rumenapf TH, Indik S. Single amino acid substitution (G42E) in the receptor binding domain of mouse mammary tumour virus envelope protein facilitates infection of non-murine cells in a transferrin receptor 1-independent manner. Retrovirology 2015; 12: 43.
CrossRef
34. Gunzburg WH, Salmons B. Mouse mammary tumor virus mediated transfer and expression of neomycin resistance to infected cultured cells. Virology 1986; 155: 236–48.
CrossRef
35. Indik S, Gunzburg WH, Salmons B, Rouault F. Mouse mammary tumor virus infects human cells. Cancer Res. 2005; 65, 6651–9.
CrossRef
36. Salmons B, Groner B, Calberg-Bacq CM, Ponta H. Production of mouse mammary tumor virus upon transfection of a recombinant proviral DNA into cultured cells. Virology 1985; 144: 101–14.
CrossRef
37. Shackleford GM, Varmus HE. Construction of a clonable, infectious, and tumorigenic mouse mammary tumor virus provirus and a derivative genetic vector. Proc. Natl Acad. Sci. USA 1988; 85: 9655–9.
CrossRef
38. Salmons B, Moritz-Legrand S, Garcha I, Gunzburg WH. Construction and characterization of a packaging cell line for MMTV-based conditional retroviral vectors. Biochem. Biophys. Res. Commun. 1989; 159: 1191–8.
CrossRef
39. Rizvi TA, Ali J, Phillip PS et al. Role of a heterologous retroviral transport element in the development of genetic complementation assay for mouse mammary tumor virus (MMTV) replication. Virology 2009; 385: 464–72.
CrossRef
40. Indik S, Gunzburg WH, Salmons B, Rouault F. A novel, mouse mammary tumor virus encoded protein with Rev-like properties. Virology 2005; 337: 1–6.
CrossRef
41. Mertz JA, Simper MS, Lozano MM, Payne SM, Dudley JP. Mouse mammary tumor virus encodes a self-regulatory RNA export protein and is a complex retrovirus. J. Virol. 2005; 79: 14737–47.
CrossRef
42. Mertz JA, Chadee AB, Byun H, Russell R, Dudley JP. Mapping of the functional boundaries and secondary structure of the mouse mammary tumor virus Rem-responsive element. J. Biol. Chem. 2009; 284: 25642–52.
CrossRef
43. Mullner M, Salmons B, Gunzburg WH, Indik S. Identification of the Rem-responsive element of mouse mammary tumor virus. Nucleic Acids Res. 2008; 36: 6284–94.
CrossRef
44. Schambach A, Galla M, Maetzig T, Loew R, Baum C. Improving transcriptional termination of self-inactivating gamma-retroviral and lentiviral vectors. Mol. Ther. 2007; 15: 1167–73.
CrossRef
45. Venkataraman K, Brown KM, Gilmartin GM. Analysis of a noncanonical poly(A) site reveals a tripartite mechanism for vertebrate poly(A) site recognition. Genes Dev. 2005; 19: 1315–27.
CrossRef
46. Aktar SJ, Vivet-Boudou V, Ali LM et al. Structural basis of genomic RNA (gRNA) dimerization and packaging determinants of mouse mammary tumor virus (MMTV). Retrovirology 2014; 11: 96.
CrossRef
47. Mustafa F, Al Amri D, Al Ali F et al. Sequences within Both the 5´ UTR and Gag Are Required for Optimal In Vivo Packaging and Propagation of Mouse Mammary Tumor Virus (MMTV) Genomic RNA. PLoS One 2012; 7: e47088.
CrossRef
48. de Jong J, Akhtar W, Badhai J et al. Chromatin landscapes of retroviral and transposon integration profiles. PLoS Gen. 2014; 10: e1004250.
CrossRef
49. De Iaco A, Santoni F, Vannier A et al. TNPO3 protects HIV-1 replication from CPSF6-mediated capsid stabilization in the host cell cytoplasm. Retrovirology 2013; 10: 20.
CrossRef
50. Diaz-Griffero F. The Role of TNPO3 in HIV-1 Replication. Mol. Biol. Int. 2012; 2012: 868597.
CrossRef
Affiliations
Stanislav Indik
Institute of Virology,
University of Veterinary Medicine Vienna, Vienna, Austria
This work is licensed under a Creative Commons Attribution- NonCommercial – NoDerivatives 4.0 International License.