Uncovering the potential of ultra scale-down tools to enable cost-effective “Quality by Design”
Cell Gene Therapy Insights 2016; 2(4), 463-471.
10.18609/cgti.2016.057
With many cell therapy companies struggling to meet the manufacturing demands of large-scale commercial production, the industry is slowly moving towards implementation of “Quality-by-Design” principles to gain an understanding of the impact of critical process parameters on critical quality attributes of the product. However, this framework needs an upfront investment in time and resources (ideally during the discovery phase) and process and product development, which is not always an option. Ultra scale-down technologies, allow high-throughput experimentation with minimal material, providing an attractive option for cost-effective screening during the discovery phase. Given adequate characterization with respect to large-scale operations, these tools hold the potential to enable process development and application of “Quality-by-Design” principles to various cell manufacturing steps, especially those concerning downstream processing. This article discusses some of the key challenges faced in cell therapy manufacturing, and how ultra scale-down devices may be implemented as tools to address these.
Potential of cell-based therapies & challenges faced
The potential of cell and gene therapies to address previously unmet patient needs by treating and curing diseases is reflected in the growth the field has experienced in the past few decades [1]. The cell therapy industry in particular is rapidly growing and becoming increasingly important, accounting for 728 clinical trials underway by close of the second quarter of 2016 [2]. Moreover, several products have been approved and/or marketed worldwide for clinical use, to name a few: Apligraf (Organogenesis), Carticel (Genzyme BioSurgery), ChondroCelect (TiGenix NV), Lavivav (Fibrocell Technologies), Dermagraft (Advanced Tissue Sciences) (FDA Marketed Products website). Nevertheless, many small and medium companies failed, and continue to do so, when attempting to overcome the number of challenges that arise from clinical trials. Most commonly, these challenges are related to cell manufacturing.
With cell therapies, the product and process go hand-in-hand [3]. The exposure of the cells to a range of mechanical and physicochemical stresses during processing can cause internal adjustments. These adjustments can lead to DNA damage and genomic alterations [4], physiological and metabolic changes [5–7], complete lysis or programmed cell death [8] and physical changes that can cause mechanical damage [5,9–12]. Therefore manufacturing is challenging because of the biological complexity of cells, the lack of understanding of their mechanisms of action, the challenges in product characterization and the inherent variability of starting materials [13]. All of these challenges have slowed down the translation of these complex therapies to industrial processes. Thus, for cell therapy to become a commercially viable industry of its own, the development of a manufacturing process that is robust, well-defined and scalable while maintaining identity, potency, purity and viability of the final live cell product is paramount [14].
Current workflows for cell-based therapies
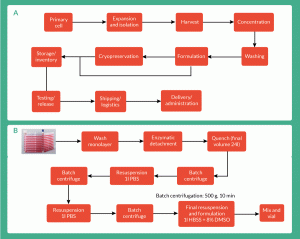
Figure 1. Process flowhseet for (A) a generic bioprocess for a whole-cell therapy (Lapinskas 2010) and (B) therapy specific manufacturing process used by Onyvax Ltd for production of cell line constituents of the Ony-P prostate cancer vaccine.
Achieving manufacturing processes as previously described, is a far from trivial affair. This is mainly because of the complexity of current workflows, the number of unit operations needed to achieve the final product and the fact that many of those are not contained and/or scalable [15].
Following upstream production (cell culture), most manufacturing processes (Figure 1) include a clarification and concentration step of the cellular material. Typically, a centrifugation or a filtration step is included to remove large aggregates, unwanted proteins, cell debris and concentrate the cellular suspension [16]. Cell-based therapies to date use batch centrifugation for cell concentration. However, batch centrifugation has proven to be a difficult step to automate while maintaining sterility [3], as it requires the use of biological safety cabinets for the transfer stages. Therefore, if these therapies are to be commercialized at an industrial scale, batch centrifugation will encounter problems when dealing with risk of contamination, large volumes and high numbers of cells [17]. The processing bottlenecks will shift to the downstream steps and will need to be addressed [16,18]. The industry faces major challenges. One of these challenges is to have the capacity downstream to manufacture and process large volumes of cells in suspension (100–1000 l) whilst achieving high cell concentration and high percentage viability within acceptable limits (limits that may vary on a case-by-case basis depending on the cell therapy in question).
Other alternatives to batch centrifugation include continuous counterflow centrifugation and tangential flow filtration, which may be more amenable to large-scale, contained and automated operations [16]. Continuous counterflow centrifugation systems, like kSep® (KBI Biopharma) and Elutra® cell separation system (Terumo BCT), can wash cells, clear residuals from the supernatant and buffer exchange, while keeping the cells in suspension. kSep® in particular can be fully automated and contained, uses a disposable flow path and can range from 0.1 to hundreds and thousands of liters. Despite its remarkable potential for cell therapies, the issue faced by companies looking to implement such technologies is the high capital investment requirement coupled with expensive disposables, which may hinder process development efforts [16]. Tangential flow filtration therefore offers an alternative that can be scaled, automated, fully contained, with a lower capital investment and costs for disposables that are more amenable to process development [16].
With any choice of technology, it must be characterized to ensure the critical quality attributes (CQAs) of the product in question are not compromised. This should be done in a robust and structured manner, in order to establish the critical process parameters (CPPs) and understand the respective design space. This is of particular relevance with downstream processes where CPPs may be spread across multiple unit operations and potentially show interactions across unit operations [19]. A framework that can be useful in assessing parameter interactions is the “Quality-by-Design” (QbD) approach.
Introducing QbD to cell manufacturing
It has become evident to many experts in the field and authorities that there needs to be further understanding of the impact of the operating variables on the product itself [15]. For instance, in early 2000s the FDA introduced the Pharmaceutical current Good Manufacturing Practice initiative to address inefficiencies and challenges in identifying underlying cause for failures during drug manufacturing. A few years later, in 2006, this initiative was followed by a framework published by the International Conference on Harmonisation outlining the QbD framework, which establishes a systematic, holistic approach to process and product management based on scientific knowledge and risk assessment.
QbD requires a thorough understanding of the product and its process of manufacture. To further define ‘thorough understanding’, it means to comprehend the impact on the product due to the variability in raw materials, the relationship between the CPPs and the product’s CQAs. Based on the number of variables that have an impact on the manufacturing of cell-based therapies, it is clear that to investigate all of the above, there is an inherent need for an investment in time and resources upfront during the discovery, process and product development phases [20]. This may be a luxury many small and medium companies cannot afford, yet may prove to be key for the successful implementation of QbD principles and thus, the development of a robust process with adequate design space. Lipsitz et al. defined QbD as “a product development and life cycle management framework promoted by regulatory agencies, and, where necessary, by investing in new technological solutions to enable its implementation” [21]. Moreover, they recognized that there are a number of opportunities to incorporate QbD into cell therapy manufacturing, yet they believe additional technology development is needed for full implementation. Although the rationale that concerns the need for technology development presented by Lipsitz et al. refers to measurable molecular and cellular characteristics of a cell population to final product quality, it is proposed here to extend the rationale to incorporate, ultra scale-down (USD) tools as part of the technological solutions needed to enable cost-effective QbD implementation.
USD technologies
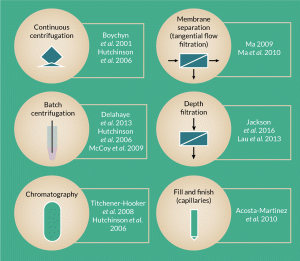
Figure 2. Schematic of ultra scale-down tools reported in literature as predictive tools for several different large-scale downstream processing steps and/or processing parameters.
Efforts have been made to develop early stage, low-cost process development predictive tools that can accurately mimic the performance of large-scale equipment using small amounts of material [22]. Historically, scaling down any process meant using a pilot plant or few liters scale. However, these options remain costly because of the need for large amounts of biological material [23]. More recently, USD tools have been developed to cover an array of techniques within cell bioprocessing and Titchener-Hooker et al. presents an overview of these techniques [22].
USD devices have been investigated as predictive tools for several different large-scale bioprocessing steps (Figure 2), such as chromatography columns [11,22,24,25], continuous centrifugation [24,26], depth filtration [26,28] and membrane separation [9,30]. Some have been shown to follow a straight forward correlation to large scale, for example, Hutchinson et al. combined the use of a rotating disc device and a laboratory-scale test tube centrifuge to successfully predict the separation characteristics of industrial scale disc stack centrifuges and investigated the effect of operating with different feed zones using milliliter quantities of biological material [24].
Owing to the small volumes of material needed, high-throughput experimentation is possible when using USD devices and therefore the scope for investigation is large. This feature is of particular importance for the cell-based industry, where the cellular material is expensive to produce. Therefore, identifying and understanding both mechanical and biological responses of candidate cells to processing stimuli at an early stage will allow the design of robust bioprocesses. This will enable the delivery of reproducible and efficient therapies, rejection of inappropriate candidates at early stages (prior to clinical manufacturing) and application of QbD principles from an early stage, as Delahaye et al. [30] and Acosta-Martinez et al. have shown [5].
Recent studies have used USD tools to investigate the impact on surface markers, growth rates and retention of membrane integrity of varying processing conditions during downstream operations for cell-based therapies [5,12,25]. With little material, one can significantly increase the throughput of the experimental phase in a cost-effective and time-efficient manner. However, for these studies to be of value, thorough characterization must be carried out to ensure there is a true relationship between the operating conditions studied in the USD tools, to those experienced during large-scale processing.
In this article, a modified version of the membrane separation USD device designed by Ma et al. was used to develop a novel methodology to investigate the effect of membrane processing on the recovery of cells for therapy.
USD membrane separation device
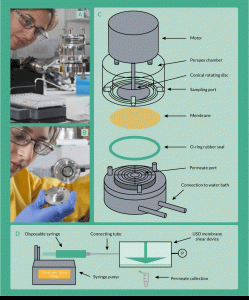
Figure 3. The ultra scale-down membrane filtration device set-up. (A) Attached to a syringe pump and (B) inside of the chamber, (C) schematic representation of the device and (D) schematic representation of full set-up of the device, including pressure measurement and syringe pump.
Several attempts to mimic the processing environment during filtration have been reported by various authors. Chandler and Zydney [31] published work on membrane screening using a small-scale high-throughput multi-well filtration plate that allows the user to simultaneously test 96 different conditions. Ghosh and Cui [33] proposed for the first time an ultrafiltration rotating disc device of ~15 mL capacity. This device consisted of a magnetically driven flat impeller that constantly stirs the retentate chamber. Based on this model, Ma et al. [29] designed a USD device with a rotating disc to mimic cross-flow microfiltration with ~10-fold less working volume than the one Ghosh and Cui had presented (volume of the retentate is ~1.7 mL) [33].
An optimized version of the latter USD device (fabricated at the Rapid Design and Fabrication Facility, in the Department of Biochemical Engineering at University College London) was used in this study (Figure 3). The most important parameter of this device is the shear rate at the membrane surface, parameter that is normally responsible for the rate of fouling of the membrane. The device was designed such that it can be run in dead end mode but mimicking shearing conditions from a cross-flow model. This feature allowed the decoupling of shear rate and pressure drop.
Using this tool, a device and methodology were developed for the characterization of the response of a human cell line to membrane-based processing, using just a small quantity of cells that is often all that is available at the early discovery stage. A model cell line for a typical adult mesenchymal cell population, which is a surrogate for commercially relevant cell lines, was used to develop the measurements. The impact was evaluated by cell damage on completion of membrane processing as assessed by trypan blue exclusion and release of intracellular lactate dehydrogenase (LDH). Dye exclusion techniques are fairly inexpensive, fast and reliable methods for cell viability measurements. In recent years, other methods, such as cytometric techniques, have gained popularity over trypan blue, mainly due to accuracy of measurement.
Overall findings using the USD membrane separation device
Similar insight was gained from both methods (trypan blue exclusion and LDH) and this allowed the extension of the use of the LDH measurements to examine cell damage as it occurs during processing by a combination of LDH appearance in the permeate and mass balancing of the overall operation. The method developed was then used to test the impact of varying operating conditions and cell lines on cell damage and morphological changes.
Characterization of desirable traits, including low rates of both cell death and loss of membrane integrity, of two clinically relevant cell lines was therefore achieved. The effects of energy dissipation rates, cell concentration (viscosity) and cell ageing prior to processing were investigated on the above mentioned CQAs.
What’s next for USD?
USD tools have the potential to add tremendous value to the regenerative medicine field by allowing cost-effective product and process development, as long as these tools are adequately characterized to mimic large-scale operations. The potential for these devices as screening tools for cell line selection and operating parameters can facilitate more in-depth understanding of the product and the process, which is paramount for cell manufacturing. For instance, for the membrane separation device described in this article, efforts going forward should ideally focus on establishing a correlation between the USD device and large-scale TFF operation. Unlocking the potential of these tools by adequate characterization to large-scale unit operations, may finally unlock the potential to applying QbD principles to cell manufacturing.
Financial & competing interests disclosure
The author has no relevant financial involvement with an organization or entity with a financial interest in or financial conflict with the subject matter or materials discussed in the manuscript. This includes employment, consultancies, honoraria, stock options or ownership, expert testimony, grants or patents received or pending, or royalties. No writing assistance was utilized in the production of this manuscript.
Acknowledgements
The author is thankful for support from: the BBSRC support for FM, the supervisory team at UCL (Prof. M Hoare, Dr I Wall, Dr K Lawrence) and the Regenerative Medicine Bioprocessing Group at UCL Biochemical Engineering (Prof. C Mason, Dr L Ruban, Dr N Jaccard and Dr S Gerontas).
References
1. Coopman K, Medcalf N. From production to patient : challenges and approaches for delivering cell therapies. The Stem Cell Research Community, StemBook, 2014; 44(0).
2. Alliance of Regenerative Medicine, 2016. Quarterly Data Report Q2 2016.
3. Mason C, Hoare M. 2006. Regenerative medicine bioprocessing: the need to learn from the experience of other fields. Regenerative Medicine 1(5). CrossRef
4. Jacobs K, Zambelli F, Mertzanidou A et al. Higher-Density Culture in Human Embryonic Stem Cells Results in DNA Damage and Genome Instability. Stem Cell Reports 2016; 6(3): 330–41. CrossRef
5. Acosta-Martinez JP, Papantoniou I, Lawrence K, Ward S, Hoare M. Ultra scale-down stress analysis of the bioprocessing of whole human cells as a basis for cancer vaccines. Biotechnol. Bioeng. 2010; 107(6): 953–63.
https:/doi.org/10.1002/bit.22888
6. Al-Rubeai M, Singh RP, Emery AN, Zhang Z. Cell cycle and cell size dependence of susceptibility to hydrodynamic forces. Biotechnol. Bioeng. 1995; 46(1): 88–92. CrossRef
7. Zoro BJH, Owen S, Drake RAL, Hoare M. The impact of process stress on suspended anchorage-dependent mammalian cells as an indicator of likely challenges for regenerative medicines. Biotechnol. Bioeng. 2008; 99(2): 468–74. CrossRef
8. Mollet M, Godoy-Silva R, Berdugo C, Chalmers JJ. 2007. Acute hydrodynamic forces and apoptosis: a complex question. Biotechnology and Bioengineering 98(4):772-88. CrossRef
9. Ma G. 2009. Development of ultra scale-down shear filtration system and modelling of large scale diafiltration system. London: University College London.
10. Mardikar SH, Niranjan K. 2000. Observations on the shear damage to different animal cells in a concentric cylinder viscometer. Biotechnology and Bioengineering 68(6):697-704. <a href=”https:/doi.org/10.1002/(SICI)1097-0290(20000620)68:63.0.CO;2-6″ target=”_blank”>CrossRef
11. McCoy R, Hoare M, Ward S. 2009b. Ultra scale-down studies of the effect of shear on cell quality; Processing of a human cell line for cancer vaccine therapy. Biotechnology Progress 25(5):1448-58. CrossRef
12. McCoy R, Ward S, Hoare M. 2010a. Sub-population analysis of human cancer vaccine cells–ultra scale-down characterization of response to shear. Biotechnology and Bioengineering 106(4):584-97. CrossRef
13. Bravery CA, Carmen J, Fong T et al. Potency assay development for cellular therapy products: An ISCT* review of the requirements and experiences in the industry. Cytotherapy 2013; 15(1): 9–19. CrossRef
14. Carmen J, Burger SR, McCaman M, Rowley JA. 2012. Developing assays to address identity, potency, purity and safety: cell characterization in cell therapy process development. Regenerative Medicine 7(1):85-100. CrossRef
15. Campbell A, Brieva T, Raviv L et al. Concise Review: Process Development Considerations for Cell Therapy. Stem Cells Transl. Med. 2015; 4(10): 1155–63. CrossRef
16. Pattasseril J, Varadaraju H, Lock L, Rowley JA. Downstream Technology Landscape for Large-Scale Therapeutic Cell Processing. BioProcess International 2013; 11(3).
17. Lapinskas E. 2010. Scaling Up Research to Commercial Manufacturing. Chemical Engineering Progress 106(11):44-49.
18. Rowley J, Abraham E, Campbell A, Brandwein H, Oh S. Meeting lot-size challenges of manufacturing adherent cells for therapy. BioProcess International 2012; 10: 16–22.
19. Meitz A, Sagmeister P, Langmann T, Herwig C. An Integrated Downstream Process Development Strategy along Quality-by-Design Principles. J. Bioeng. 2014; 1(4): 213–30.
20. Rathore AS, Winkle H. Quality by design for biopharmaceuticals. Nature Biotechnol. 2009; 27(1): 26–34.
CrossRef
21. Lipsitz Y, Timmins NE, Zandstra PW. Quality cell therapy manufacturing by design. Nature Biotechnol. 2016; 34(4): 393–400.
CrossRef
22. Titchener-Hooker NJ, Dunnill P, Hoare M. Micro biochemical engineering to accelerate the design of industrial-scale downstream processes for biopharmaceutical proteins. Biotechnol. Bioeng. 2008; 100(3): 473–87. CrossRef
23. Tait AS, Aucamp JP, Bugeon A, Hoare M. Ultra scale-down prediction using microwell technology of the industrial scale clarification characteristics by centrifugation of mammalian cell broths. Biotechnol. Bioeng. 2009; 104(2): 321–31.
https:/doi.org/10.1002/bit.22393
24. Hutchinson N, Bingham N, Murrell N, Farid S, Hoare M. 2006. Shear stress analysis of mammalian cell suspensions for prediction of industrial centrifugation and its verification. Biotechnology and Bioengineering 95(3):483-91. CrossRef
25. Delahaye M. An ultra scale-down study of recovery by centrifugation of human cells for therapy. University College London, UK (2013).
26. Boychyn M, Yim S, Ayazi Shamlou P, Bulmer M, More J, Hoare M. Characterization of flow intensity in continuous centrifuges for the development of laboratory mimics. Chem. Eng. Sci. 2001; 56(16): 4759–70. CrossRef
27. Jackson NB, Liddell JM, Lye GJ. An automated microscale technique for the quantitative and parallel analysis of microfiltration operations. J. Membrane Sci. 2006; 276, 31–41. CrossRef
28. Lau EC, Kong S, McNulty S et al. An ultra scale-down characterization of low shear stress primary recovery stages to enhance selectivity of fusion protein recovery from its molecular variants. Biotechnol. Bioeng. 2013;110, 1973–83. CrossRef
29. Ma G, Aucamp J, Gerontas S, Eardley-Patel R, Craig A, Hoare M, Zhou Y. 2010. Mimic of a large-scale diafiltration process by using ultra scale-down rotating disc filter. Biotechnology Progress 26(2), 466–76.
30. Delahaye M, Lawrence K, Ward S, Hoare M. An ultra scale-down analysis of the recovery by dead-end centrifugation of human cells for therapy. Biotechnol. Bioeng. 2014; 112(5), 997–1011. CrossRef
31. Chandler M, Zydney A. High throughput screening for membrane process development. Membrane Science 2004; 237(1), 181–188. CrossRef
32. Delahaye M, Lawrence K, Ward S, Hoare M. An ultra scale-down analysis of the recovery by dead-end centrifugation of human cells for therapy. Biotechnol. Bioeng. 2014; 112(5): 997–1011. CrossRef
33. Ghosh R, Cui Z. Analysis of protein transport and polarization through membranes using pulsed sample injection technique. Membrane Science 2000; 175(1), 75–84. CrossRef
Affiliation
Fernanda Masri
Development Engineer at the Centre for Commercialization of Regenerative Medicine (CCRM). This work was carried out in University College London.
This work is licensed under a Creative Commons Attribution- NonCommercial – NoDerivatives 4.0 International License.