Advances and challenges of successful cell therapies for liver disease
10.18609.cgti.2015.011
Review
End-stage liver disease is a common cause of morbidity and mortality. Currently, the gold standard treatment is orthotopic liver transplant; however, the mismatch between organ demand and supply, and the risks associated with organ transplantation has led to a search for alternative therapies. Cellular therapies could potentially present such an alternative to transplant or bridge to transplant. The earliest work has involved transplantation of allogeneic hepatocytes, with modest benefits shown. Hepatic progenitor cells have also been used in experimental settings, but cellular engraftment is challenging. Use of embryonic stem cells and pluripotent stem cells to form hepatocyte-like cells has also been investigated. Various bone marrow cells have shown therapeutic potential, likely via paracrine effects on liver repair and regeneration. This review summarizes the key advances in cellular therapies for liver disease, and discusses the challenges that need to be overcome before these therapies can be translated into clinical practice.
Liver disease is a common cause of morbidity and mortality worldwide and causes include acute and chronic liver failure along with inherited metabolic diseases [1]. Currently the only effective treatment for advanced liver disease is orthotopic liver transplantation (OLT). However, demand for organs exceeds supply, with approximately 20% of patients dying whilst on the transplantation waiting list [2]. Furthermore, liver transplantation is associated with major surgical risks, with many patients being insufficiently fit for transplant due to co-morbidities and the debilitating effects of liver disease. Moreover, after transplantation, patients are required to take life-long immunosuppression, which has numerous side effects and can impact quality of life.
Auxiliary partial OLT has been used successfully to treat certain metabolic liver diseases and acute liver failure [3], suggesting that the functions of the liver can be performed without the whole organ. This has prompted research into novel cellular therapies for the treatment of liver disease.
Cellular therapies could potentially be used as an alternative to OLT, or as a bridge to transplant. They offer potential logistical and safety advantages over whole-organ transplant as summarized in Box 1: they are usually administered by intravascular catheters, so are less invasive than OLT, with lower immediate morbidity and mortality. For hepatocyte transplantation the same organ can provide cells to multiple recipients, thereby improving the ratio of donors to recipients on the waiting list. Currently, many donated livers are not accepted for OLT, yet these livers could be used to provide therapeutic cells. Another advantage is that cellular therapies do not necessitate the removal of the native liver, which in the setting of acute liver failure would provide the native liver with an opportunity to recover.
Summary of some of the key advantages of cellular therapies over orthotopic liver transplant (OLT). |
---|
Less invasive |
May utilize organs unsuitable for OLT |
One donor can supply cells to multiple recipients |
The native liver can remain in situ |
Lower financial costs |
In selecting a cell therapy for a patient with liver disease, it is important to have an understanding of the underlying disease process, as the requirements may be very different. Inherited metabolic diseases are usually characterized by a single enzymatic defect within the hepatocyte, and therefore replacement with functional allogeneic hepatocytes would be therapeutically effective. Chronic liver failure is usually associated with liver cirrhosis, which is characterized by deposition of extra-cellular matrix proteins throughout the liver, alteration in the hepatic architecture and loss of hepatic parenchymal cells. This commonly results in impaired liver function, and the clinical need is restoration of synthetic function and reduction in liver fibrosis. Resolution of fibrosis would depend on either apoptosis of the myofibroblast cells which synthesize the fibrous tissue, or enzymatic breakdown of the fibrous tissue by matrix metallic proteinases (MMP) [4]. In acute hepatic inflammation, as seen in autoimmune liver disease, there is a need to dampen inflammation and thus cells with immunomodulatory properties, such as regulatory T or mesenchymal stromal cells, are required.
This review article provides an overview of the advances in cellular therapies for the treatment of liver disease, as well as highlighting some of the current challenges associated with their use.
Options for cellular therapies
Some cellular therapies in liver disease aim for homologous reconstitution of the native liver; however, the seeding of healthy hepatocytes may not always be necessary to treat liver disease. In rats it was shown that the use of cultured supernatant from cells and fragmented cells was beneficial in the setting of acute liver failure [5]. Therefore, other cell types that can influence cell generation and repair are additional candidates for cell therapies.
Homologous reconstitution of liver cells
Hepatocyte transplantation
Due to limited organ availability, hepatocytes are usually sourced from whole livers considered unsuitable for OLT, which often means cell quality is poor. Currently, around 20–40% of donated organs are discarded [6]. Traditionally, cold static preservation of organs has been used, which is associated with ischemia–reperfusion injury; however, more recent methods of organ preservation, such as the use of enhanced machine perfusion devices, may allow greater utilization of marginal organs, and thus increase the number of transplantable liver organs as well as those that can be used for liver cell isolation [7,8].
Cells can be cryopreserved after isolation; however, repeated freeze–thaw cycles can compromise cells’ metabolic function. Methods of reducing this damage are being investigated, such as the use of vitrification to prevent crystallization of cells, and encapsulation of hepatocytes prior to cryopreservation to provide mechanical protection. However, these protocols require validation and refinement [9–11].
Normal hepatocytes from allogeneic donors can be used to provide missing gene products in inherited metabolic diseases, as demonstrated in animals and humans with Criggler-Najjar syndrome, urea cycle disorders, and familial hypercholesterolemia [12–15]. Hepatocyte transplantation has also been undertaken in acute and chronic liver failure [16–19] where the goal is to restore parenchymal cell mass and synthetic function, although in most cases, the effects have been modest and of uncertain duration [20]. Although fetal hepatocytes have been shown to demonstrate anti-fibrotic properties in rats, ethical concerns have thus far restricted their use to animal experiments [21].
Until recently, it was difficult to measure and track transplanted hepatocytes. However, new non-invasive methods are being developed, such as MRI detection of hepatocytes labelled with iron oxide nanoparticles [22]. The challenge is to identify markers that are safe to patients, do not compromise function/engraftment of infused cells and are readily visualized.
Currently, one of the major limitations of hepatocyte transplantation is limited availability of good quality cells. In an attempt to overcome problems of cell shortages, research has included methods of immortalizing cells, the use of xenogeneic hepatocytes, and the use of autologous cells. Gene transfer from the simian virus 40 (SV40) to hepatocytes can immortalize them, although there are concerns about the transfer of malignancy to the host [23]. Use of xenogeneic hepatocytes from pigs has been explored, although immunogenic differences between pigs and humans can result in hyper-acute rejection due to xenoreactive antibodies against components of the porcine endothelium (such as the Galal-3Galp1–4G1cNAc oligosaccharide) [24]. Genetic knockout of these antigens can reduce the risk of immune rejection [25], although there still remains a risk of transferring zoonotic diseases, such as porcine endogenous retroviruses (PERV), to patients [26]. Use of autologous cells would reduce the problem of cell shortage and avoid the need for immunosuppression. In inherited metabolic disease, this requires prior correction of the genetic defect. In humans with familial hypercholesterolemia, where the defective LDL receptor was genetically modified before re-implantation, there was a mild, albeit not clinically significant, reduction in serum cholesterol [19]. An alternative means of avoiding immunosuppression could be to genetically modify host or donor cells to prevent immune rejection, by for example introduction of immunomodulatory genes into donor hepatocytes before transplantation [27].
Another challenge is that there is difficulty in achieving long-term hepatocyte engraftment and survival in damaged liver, which means that repeated infusions may be required. Furthermore, after hepatocyte transplantation, it is difficult to achieve sufficient in vivo proliferation. Although hepatocytes have excellent regenerative capacity, physiological mechanisms ensure that hepatocyte numbers are constant, and thus hepatocytes usually require a stimulus to proliferate. In murine models of hereditary tyrosinemia and alpha-1-antitrypsin deficiency, transplanted hepatocytes have a survival advantage over the defective endogenous cells, enabling the liver to be repopulated with healthy cells [28,29]. However, in most other inherited liver diseases, such as Criggler-Najjar syndrome, the regenerative capacity of the native hepatocytes remains normal, and suppression of native cell proliferation is needed to promote proliferation of transplanted cells. In experiments, this is often achieved by irradiation of the liver or partial hepatectomy [30,31]. Although notably fetal hepatocytes can proliferate without any stimulus, this has not been a consistent finding [32,33].
Thus, hepatocyte transplantation offers promise for the correction of specific disease processes such as urea cycle enzyme defects. However, cell availability and in vivo proliferation is a limiting factor. The challenges of engraftment in end-stage liver disease render it a more difficult target. In these cases, it may be just as important to target the surrounding environment, rather than just the hepatocytes.
Transplantation of hepatic progenitor cells
In response to mild liver injury, mature hepatocytes contribute to the majority of liver regeneration [34] whereas in severe liver disease this regenerative ability in endogenous hepatocytes is exhausted. In this setting, there is activation of endogenous liver stem cells, known as hepatic progenitor cells or oval cells, which are multipotent cells in the canals of Hering [35]. Oval cell proliferation may be induced experimentally through targeted gene deletion to suppress hepatocyte proliferation [36]. Oval cells can differentiate both into hepatocytes and cholangiocytes, and have been implicated in liver regeneration and repair [37].
Yovchev et al demonstrated that rat fetal hepatic progenitor cells, when transplanted into rats with thioacetamide-induced fibrosis/cirrhosis, repopulated the liver and exerted an antifibrotic effect [21]. Moreover, ablation of oval cells, identified using the marker fox1, was found to impair recovery from liver injury [38].
Unfortunately, hepatic progenitor cells are only available in limited numbers, and usually require significant regenerative stimulus to proliferate. There are also concerns that activation of oval cells may drive a fibrogenic response, through differentiation into cholangiocytes which secrete pro-fibrogenic factors acting on myofibroblasts and hepatic stellate cells [39]. Oval cells cannot currently be delivered as cell suspensions for use in clinical trials, and therefore have not been tested. Interestingly, use of G-CSF has been associated with clinical improvement in patients with chronic liver disease [40]. Oval cells express a G-CSF receptor, and levels of oval cell mobilization and proliferation have shown to increase following G-CSF infusion [41]. The underlying mechanism of G-CSF action may be stimulation of endogenous repair.
Transplantation of embryonic stem cells
Embryonic stem cells (ESCs) are pluripotent cells derived from the blastocyst, approximately 5 days following fertilization. However, their extraction requires destruction of the blastocyst, thus presenting ethical and legal concerns to their use, which limits their clinical application.
Using the appropriate culture medium, they can be maintained in an undifferentiated state and directed to differentiate into every cell type, including hepatocytes [42]. Takayama et al used two transcription factors, FOXA2 and HNF1α, to induce differentiation of human ESCs into hepatocyte-like cells, which were functionally similar to hepatocytes producing albumin and urea, taking up indocyanine green, and metabolizing drugs [43]. Transplantation of ESC-derived hepatocytes in a mouse model of acute CCl4 injury increased host hepatocyte proliferation and revascularization, whilst reducing alanine aminotransferase levels [44]. ESCs thus potentially provide an infinitely expandable source of hepatocyte-like cells. As yet however, these cells are more similar to fetal hepatocytes than adult hepatocytes when differentiated in vitro and thus further refinements to differentiation protocols are needed.
Direct injection of undifferentiated ESCs causes the formation of teratomas containing hepatocyte-like cells, which creates an unacceptable cancer risk and thus restricts their use. No teratomas were found when ESCs were induced to differentiate into hepatocyte-like cells prior to transplantation, although further longer-term studies are required.
Transplantation of induced pluripotent stem cells
Induced pluripotent cells (iPSCs) were first generated in 2006 [45]. They are somatic cells that have undergone reprogramming to adopt a phenotype similar to embryonic stem cells. This reprogramming is achieved using transcription factors, such as Sox2, KIf4 and c-myc, and iPSCs can be generated from almost any tissue type. Human iPSCs can therefore be induced to differentiate into hepatocyte-like cells, in a process similar to differentiation of ESCs [45]. iPSCs avoid ethical issues associated with use of ESCs, and can potentially be used autologously, removing the need for immunosuppression.
iPSC-derived hepatocyte-like cells were able to engraft and proliferate after transplantation into fumarylacetoacetate hydrolase knockout mice (FAH-/-) mice [46]. Notably iPSCs from patients with alpha-1-antitrypsin deficiency were genetically modified to correct the defect, and were, after successful differentiation in vitro to hepatocyte-like cells, able to repopulate recipient murine liver with alpha-1-antitrypsin-positive cells [47].
Hepatocyte-like cells produced from iPSCs may also be used for creating patient-specific in vitro disease models, so called ‘disease in a dish’. Furthermore they could be used for drug testing, for both toxicity and therapeutic assessment as for example has been tried in familial hypercholesterolemia where cells demonstrated an increase in LDL uptake in response to lovastatin [48]. They have also been used to model alpha-1-antitrypsin deficiency and hereditary tyrosinemia [49].
There are still differences in the gene expression and functionality of hepatocytes produced from iPSCs and primary hepatocytes [50]. The ‘hepatocyte-like cells’ generated from iPSCs are more similar to fetal hepatocytes than adult hepatocytes, for example because they express alpha fetoprotein, and demonstrate embryonic p450 activity [51]. Following transplantation, hepatocytes from iPSCs do not proliferate as effectively as primary hepatocytes [52]. Furthermore, there remain concerns about the long-term sequelae of genetic reprogramming, and the potential for malignant transformation of cells.
Transplantation of directly programmed cells
In experimental studies, fibroblasts can be directly programmed into hepatocytes with use of specific transcription factors, Hnf4alpha plus Foxa1 [50]. By ‘bypassing’ the pluripotent stage, there is a reduced risk of malignant transformation with these cells.
In a recent study, fibroblasts were programmed directly into a readily expandable pool of multipotent progenitor cells, which then differentiated into hepatocytes [53]. When transplanted into a model of FAH-/- mice, these cells demonstrated higher rates of repopulation compared to cells derived from iPSCs, although still lower than that of adult hepatocytes.
Transplantation of cells that modulate liver regeneration & repair
Experimental and clinical studies suggest that infusion of bone marrow cells can modulate liver fibrosis [54–56]. Some studies have found an improvement in fibrosis following infusion of unsorted bone marrow cells, whilst others found no effect, and in some, there was worsening of fibrosis [57–60]. Bone marrow contains a mixture of cells, including hematopoietic stem cells, mesenchymal stem cells (MSCs) and macrophages and using such mixed populations limits our understanding of their mechanisms of action. Therefore several studies have attempted to isolate the various bone marrow cell populations to study them individually.
Macrophages in liver disease
Macrophages are derived from monocytes, and can play a role in both progression and regression of fibrosis [61]. There are different subsets of monocytes and macrophages, which differ in their expression of adhesion molecules and chemokine receptors, for example, human monocytes can be defined by differential expression of CD14 and CD16, whereas the corresponding murine markers are Ly6Chi and Ly6Clo.
Ruhnke et al demonstrated that monocytes can differentiate into hepatocyte-like cells after treatment with macrophage colony stimulating factor/interleukin (IL)-3 and culture in hepatocyte medium [62]. The resulting cells expressed specific hepatocyte markers and demonstrate functional similarity to hepatocytes. Most evidence suggests that their main action is likely mediated through paracrine effects.
Thomas et al administered bone marrow-derived macrophages in CCl4-induced murine liver fibrosis and demonstrated increased albumin production and reduced liver fibrosis with an associated upregulation of MMP enzymes [60]. Administered macrophages also promoted recruitment of host macrophages, thereby achieving an amplified response. Ramachandran et al found that different monocyte subsets were present at different time-points in liver injury; soon after injury there was a predominance of Ly6Chi monocytes associated with inflammation, whilst at maximal scar resolution there were more Ly6Clo monocytes. These Ly6Clo monocytes were associated with increased expression of MMP, growth factors and phagocytosis-associated genes. Macrophage depletion using mice transgenic for the CD11b promoter diphtheria toxin receptor caused preferential depletion of Ly6Clo monocytes, and persistence of fibrosis in mice [63].
Mononuclear cells in liver disease
Bone marrow mononuclear cells include MSCs, hematopoietic stem cells, endothelial progenitor cells and stromal cells. Six clinical studies have been conducted – of which three were randomized controlled trials – where a single dose of bone marrow mononuclear cells was administered to patients with chronic liver disease [64–69]. Five of the trials demonstrated improvement in liver indices, such as serum albumin, total protein and Child-Pugh score, although the largest trial, with 28 patients in the treatment arm, showed no significant difference [69]. However, they were all small unpowered trials, and possible explanations for discrepancies in their results include different routes of administration, different rates of relapse in patients with alcohol-induced liver disease and different doses of cells infused.
MSCs in liver disease
MSCs are multipotent stem cells that can differentiate into cells of mesodermal tissues, such as bone, muscle, cartilage, fat and neural tissue [70], and can be isolated from a range of tissue sources, including bone marrow, fat and umbilical cord. As umbilical cord MSCs are more abundant than bone marrow, they are proving to be a more regular source of MSCs.
Tanimoto et al demonstrated that infusion of 5 x 105 bone marrow-derived MSCs significantly improved fibrosis in the nonobese diabetic/severe combined immunodeficiency mouse exposed to CCl4 injury [71]. Similar results were obtained in further murine studies, with repeated infusions being more beneficial than a single infusion [72,73].
A number of clinical trials have utilized MSC therapy in liver disease, including two pilot studies, five randomized controlled trials, and several non-randomized controlled trials [74–83]. In all but one, there were some beneficial effects of MSC infusion, including improvement in model for end-stage liver disease (MELD) score, quality of life, and albumin level for example, although there was no significant change in patient survival. There were no significant side effects and the therapy was well tolerated. Histological evaluation was only performed in two studies, which demonstrated histological improvement following cell transplantation in patients with liver cirrhosis [81,82]. Different doses of cells and routes of administration used in the trials may partially explain the variability in results.
MSCs can differentiate into hepatocytes; however, it is more likely that their immunomodulatory and anti-fibrotic effects will be most relevant. Adult MSCs can be induced to differentiate into hepatocyte-like cells in vitro and in vivo under the influence of hormones, cytokines, growth factors and other cells [84–86]. Luk et al describe differentiation of rat MSCs into hepatocytes following co-culture with hepatocytes, even in the absence of additional growth factors [87]. Rabani et al infused MSCs in mice with CCl4-induced liver injury [88] and demonstrated a subsequent reduction in liver fibrosis, even though relatively few of the cells were found to engraft in the liver. Therefore, paracrine mechanisms are more likely to be responsible for the beneficial effects.
MSCs have immunomodulatory properties, which can influence cells responsible for liver injury, and protect hepatocytes from cell death. For example, prostaglandin E2 made by MSCs reduces production of pro-inflammatory cytokines such as TNFa by dendritic cells, Th1 and Th2 cells, and increases production of anti-inflammatory cytokines such as IL-10 from dendritic cells [89,90]. Prostaglandin E2 also stimulates proliferation of the immunomodulatory T-reg cells, and reduces cytotoxicity of NK cells [91]. Through downregulation of co-stimulatory molecules, C80 and CD86, on antigen presenting cells, MSCs can render T-cells anergic [89]. Xagorari et al demonstrated that hepatocytes in MSC-conditioned medium were relatively protected from CCl4-induced apoptosis, mediated through increased IL-6 production [92].
MSCs exert anti-fibrotic effects, potentially mediated in part by release of IL-10, which reduces collagen deposition and increases expression of MMPs to aid resolution of scar tissue [93,94]. Furthermore, MSCs induced hepatic stellate cell apoptosis in co-culture, through the release of hepatocyte growth factor (HGF) and nerve growth factor [95].
Due to their multiple actions, the utilization of MSCs has been proposed for a range of liver diseases including liver cirrhosis, liver failure and immune-mediated liver diseases, although there are concerns that MSCs may give undergo unwanted differentiation into myofibroblasts, which is more likely to occur if they are injected during the acute liver injury rather than the “resolution phase” [96]. Allogeneic MSC transplantation brings with it risks of immune rejection, and transfer of viruses such as cytomegalovirus and herpes simplex virus [97]. Allogeneic MSCs can also trigger an immune response, leading to rejection of the transplanted cells and hence poor engraftment.
Hematopoietic stem cells
HSCs are multipotent cells, characterized by the expression of cell-surface markers CD34 and CD133, and which have the ability to differentiate into all cells of hematopoietic lineage.
A number of clinical studies have utilized purified HSCs. Autologous infusions of HSCs were given to patients undergoing portal vein embolisation prior to liver resection for metastatic liver cancer where immediate resection was not possible due to insufficient residual liver volume. HSC infusion was associated with a more rapid increase in liver volume, thus enabling surgery to be performed earlier [98,99].
In another early clinical study, G-CSF-mobilized peripheral blood was collected from nine patients with liver cirrhosis. CD34+ cells were selected, and reinfused into the hepatic artery, and 2 months later serum albumin increased with a reduction in bilirubin [100]. In further studies, some groups obtained peripheral blood to select HSCs whilst others obtained them by direct bone marrow aspirate. The dose and route of injection also varied, with some studies administering HSCs via a peripheral vein, and others via the portal vein or hepatic artery [101–108]. These studies suggest that HSC administration is safe and feasible, with some improvement in liver blood tests and Child-Pugh score. Follow-up studies suggest that the beneficial effects are mainly up to 1 year post infusion [103]. In a non-randomized study of 50 patients with chronic liver cirrhosis secondary to hepatitis C, Salama et al found that patients receiving stem cell therapy reported greater improvement in quality of life [109]. However, most clinical trials using HSCs have been small, uncontrolled studies, with short follow up and larger controlled trials will be needed in order to draw robust conclusions.
HSCs can engraft into tissues and participate in tissue regeneration [110–113], although the mechanisms of action remain unclear. There are three main theories:
The first is that HSCs differentiate into functional hepatocytes. When lethally irradiated female mice received whole bone marrow transplants from males, a subgroup of these cells, which were CD34+linneg, were found to engraft in the liver and up to 2.2% of the hepatocytes contained the Y chromosome [45] indicating a contribution from donor cells. However reports of transdifferentiation of bone marrow cells are variable [114,115], with some evidence suggesting that purified HSCs are more likely to transdifferentiate than other bone marrow cells. In a mouse model of hereditary tyrosinemia, injection of purified HSCs was associated with around 10% seeding in the liver tissue in clusters around the diseased areas. However, with non-HSC bone marrow cells, there was no significant engraftment in this study [110]. Jang et al injected HSCs into mice with acute CCl4 liver injury and observed an increased number of hepatocytes. Through analysis of chromosomes, and protein expression, they concluded that it was transdifferentiation and not fusion that was responsible [116]. They found that the degree of conversion of HSCs into hepatocytes was related to the degree of injury, and within 7 days normal liver function was restored.
A second theory is that there is fusion of HSCs and hepatocytes, as demonstrated in the setting of hereditary tyrosinemia, using the FAH-/- mouse. When FAH-/- mice were transplanted with FAH+/+ bone marrow cells they developed nodules containing genes from both the donor and the host [117,118], which then had a survival advantage, and thus could repopulate the liver. However, in other models, cell fusion is a rare event, and if it occurs the chimeric cells only engraft in bone marrow for a short time [116,119,120]. This is likely because there is no sustained selection pressure or survival advantage. Thus cell fusion is unlikely to explain the effects of HSCs.
The third theory, which has greater support, is that stem cells exert a paracrine effect to promote regeneration and repair, although these exact mechanisms remain undefined. HGF is upregulated during liver injury and in liver failure, and is important in proliferation and development of HSCs [121]. It may be that HSCs secrete cytokines that stimulate endogenous hepatocyte proliferation, such as IL-6, TNF-a and HGF [122,123]. When Sakaida et al infused bone marrow into mice with CCl4-induced liver injury, they demonstrated a significant reduction in liver fibrosis, associated with an increase in hepatic MMP expression [57]. Some studies suggest that donor bone marrow cells are the source of this MMP, or it may be that the HSCs help to recruit other cells which can release MMP, such as macrophages [57,60,124,125].
Translation Insight
Cellular therapies offer exciting novel treatment options for advanced liver disease, and over the last two decades there have been many advances in this field. A variety of cell therapies have shown therapeutic potential, and many are in clinical trials (Figure 1).
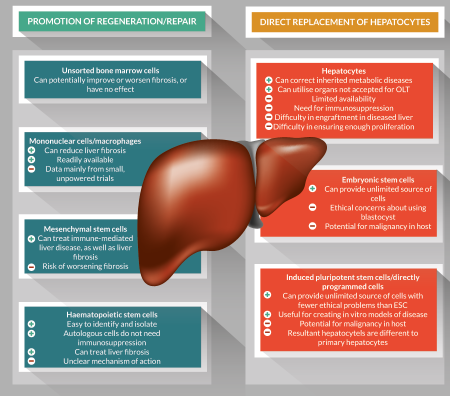
Figure 1. Summary of some of the key advantages and disadvantages of various cellular therapies for treatment of liver disease.
Differentiated hepatocytes can be transplanted, into the liver or in an extra-hepatic location, and hold promise for the treatment of inherited metabolic diseases, and short-term correction of liver failure. However, shortage of organ donors, poor engraftment and the need for immunosuppression limits their use at present. Another option is to use pluripotent stem cells, including ESCs or iPSCs, although there are concerns about their potential to become phenotypically unstable and form tumors. Future work could further investigate mechanisms of preventing this, for example, ‘suicide genes’ that can be incorporated into cells, which become activated on consuming a particular drug. The use of induced pluripotent cells offers remarkable potential for in vitro modelling of human diseases to understand pathogenesis and response to treatments.
Alternative approaches using bone marrow-derived cells, including mononuclear cells/macrophages, MSCs and HSCs, which are promising due to their putative properties in promoting regeneration and repair. Specifically, they may be of particular use in reversing the distorted liver architecture in chronic liver disease, and several small trials have shown that this approach is safe and feasible. Larger trials with standardized treatment protocols will be required to draw robust conclusions.
As cellular therapies for liver disease are still a relatively new concept, much remains to be determined regarding optimizing cellular transplantation protocols. For example, there is a need to determine:
1. Best methods for cell isolation
2. Optimal number of cells/ infusions required for transplant – further work should determine the number of cells per infusion and how many infusions are necessary for optimal effect. Research is in progress to find methods of delaying cell clearance, for example using encapsulation of hepatocytes in alginate beads to prevent exchange of antibodies and immune cells [126].
3. Best route of delivery – hepatic or splenic artery infusion is associated with a risk of embolic complications and cell damage from shear stress [127]. Hepatic artery infusion has been associated with artery dissection, Tako-Tsubu syndrome and one cause of radiocontrast nephropathy causing fatal hepatorenal syndrome [101,128]. Portal vein injection is associated with a rise in portal pressures, which can limit the numbers of cells administered at any given time.
4. Methods of improving engraftment – cell transplantation initiates an inflammatory response, causing release of molecules to make the endothelial lining more permeable. The transplanted cells must then adhere to and migrate through the sinusoidal endothelium into the liver sinusoids. Subsequent integration into the liver parenchyma depends on physical joining of the transplanted and native hepatocytes, mediated by MMP enzymes [129]. Only 10–20% of transplanted hepatocytes actually integrate into the tissue. This could be due to damage from the inflammatory response, or a failure to adhere to sinusoidal endothelium for example. Researchers have investigated ways of improving engraftment. For example, donor cells may be modified to include extracellular matrix components to aid adhesion to the liver sinusoidal endothelial cells [130]. Pre-treatment of rats with a TNF-a antagonist, etanercept, dampened the inflammatory response to hepatocyte transplantation, prolonging cell survival [131]. Agents such as doxorubicin can be given to disrupt the hepatic endothelial barrier permitting easier passage of transplanted cells.
5. Methods for tracking cells – genetically labelled probes that are often used in animal studies are not safe for use in humans. Short-term cell tracking, for example to assess cell distribution, can be achieved using indium-111 and 99m technetium. However, further work is needed to find the best way of assessing the fate of cells over a longer period.
6. The optimal immunosuppression regime – allogeneic cell transplantation produces an immune response which can lead to rapid cell clearance. Further work should look into the best choice of immunosuppressive agents to prevent this happening. Ideally, cellular therapies should remove the need for immunosuppression all together.
Conclusion
Cellular therapies have the potential to transform the future management of acute and chronic liver diseases. Although experimental work and small clinical trials suggest therapeutic benefit, the underlying mechanisms are uncertain. Further work needs to establish practical aspects of how the cell therapy should be delivered, and ideally to remove the need for immunosuppression. In the use of stem cells, the theoretical risk of malignancy needs to be researched and minimized further. A greater understanding of the underlying mechanisms of various cell therapies is crucial to tailoring the cell therapy for specific disease processes.
Once the above issues are addressed and tested in large randomized, controlled trials with long-follow up periods, cellular therapies have the potential to revolutionize the management of advanced liver disease.
Financial disclosure
The authors have no relevant financial involvement with an organisation or entity with a financial interest in or financial conflict with the subject matter or materials discussed in the manuscript. This includes employment, consultancies, honoraria, stock options or ownership, expert testimony, grants or patents received or pending, or royalties.
No writing assistance was utilised in the production of this manuscript.
Affiliations
Reenam S Khan
National Institute for Health Research Liver Biomedical Research Unit and Centre for Liver Research, University of Birmingham, Birmingham, UK
Professor Philip N. Newsome*
*Author for Correspondence
Professor of Hepatology, NIHR Birmingham Liver BRU and Centre for Liver Research, University of Birmingham, Birmingham, UK, B15 2TH.
Email: P.N.Newsome@bham.ac.uk
This work is licensed under a Creative Commons Attribution- NonCommercial – NoDerivatives 4.0 International License.
References
1. Lim YS & Kim WR. The global impact of hepatic fibrosis and end-stage liver disease. Clin. Liver Dis. 2008; 12, 733–46, vii.
CrossRef
2. Dienstag JL & Cosimi AB. Liver transplantation – a vision realized. N. Engl. J. Med. 2012; 367, 1483–5.
CrossRef
3. Rela M, Muiesan P, Vilca-Melendez H et al. Auxiliary partial orthotopic Liver Transplantation for Crigler-Najjar syndrome type I. Ann. Surg. 1999; 229, 565–9.
CrossRef
4. Iredale JP, Benyon RC, Pickering J et al. Mechanisms of spontaneous resolution of rat liver fibrosis. Hepatic stellate cell apoptosis and reduced hepatic expression of metalloproteinase inhibitors. J. Clin. Invest. 1998; 102, 538–49.
CrossRef
5. Makowka L, Rotstein LE, Falk RE et al. Reversal of toxic and anoxic induced hepatic failure by syngeneic, allogeneic, and xenogeneic hepatocyte transplantation. Surgery 1980; 88, 244–53.
PMid
6. Orman ES, Barritt AS, Wheeler SB, Hayashi PH. Declining liver utilization for transplantation in the United States and the impact of donation after cardiac death. Liver Transpl. 2013; 19, 59–68.
CrossRef
7. Fontes, P, Lopez R, van der Plaats A et al. Liver preservation with machine perfusion and a newly developed cell-free oxygen carrier solution under subnormothermic conditions. Am. J. Transplant. 2015; 15, 381–94.
CrossRef
8. Ravikumar R, Leuvenink H, Friend PJ. Normothermic liver preservation: a new paradigm? Transpl. Intl 2015; 28, 690–9.
CrossRef
9. Terry C, Hughes RD, Mitry RR, Lehec SC, Dhawan A. Cryopreservation-induced nonattachment of human hepatocytes: role of adhesion molecules. Cell Transplant. 2007; 16, 639–47.
CrossRef
10. Wu Y, Yu H, Chang S, Magalhaes R, Kuleshova LL. Vitreous cryopreservation of cell-biomaterial constructs involving encapsulated hepatocytes. Tissue Eng. 2007; 13, 649–58.
CrossRef
11. Mei J, Sgroi A, Mai G et al. Improved survival of fulminant liver failure by transplantation of microencapsulated cryopreserved porcine hepatocytes in mice. Cell Transplant. 2009; 18, 101–10.
CrossRef
12. Fox IJ, Chowdhury JR, Kaufman SS et al. Treatment of the Crigler-Najjar syndrome type I with hepatocyte transplantation. N. Engl. J. Med. 1998; 338, 1422–6.
CrossRef
13. Matas AJ, Sutherland DE, Steffes MW et al. Hepatocellular transplantation for metabolic deficiencies: decrease of plasms bilirubin in Gunn rats. Science 1976; 192, 892–4.
CrossRef
14. Horslen SP, McCowan TC, Goertzen TC et al. Isolated hepatocyte transplantation in an infant with a severe urea cycle disorder. Pediatrics 2003; 111, 1262–7.
CrossRef
15. Meyburg J, Das AM, Hoerster F et al. One liver for four children: first clinical series of liver cell transplantation for severe neonatal urea cycle defects. Transplantation 2009; 87, 636–41.
CrossRef
16. Kobayashi N, Ito M, Nakamura J et al. Hepatocyte transplantation improves liver function and prolongs survival in rats with decompensated liver cirrhosis. Transplant. Proc. 1999; 31, 428–9.
CrossRef
17. Demetriou AA, Reisner A, Sanchez J et al. Transplantation of microcarrier-attached hepatocytes into 90% partially hepatectomized rats. Hepatology 1988; 8, 1006–9.
CrossRef
18. Habibullah CM, Syed IH, Qamar A, Taher-Uz Z. Human fetal hepatocyte transplantation in patients with fulminant hepatic failure. Transplantation 1994; 58, 951–2.
CrossRef
19. Grossman M, Raper SE, Kozarsky K et al. Successful ex vivo gene therapy directed to liver in a patient with familial hypercholesterolaemia. Nat. Genet. 1994; 6, 335–41.
CrossRef
20. Fisher RA & Strom SC. Human hepatocyte transplantation: worldwide results. Transplantation 2006; 82, 441–9.
CrossRef
21. Yovchev MI, Xue Y, Shafritz DA, Locker J, Oertel M. Repopulation of the fibrotic/cirrhotic rat liver by transplanted hepatic stem/progenitor cells and mature hepatocytes. Hepatology 2014; 59, 284–95.
CrossRef
22. Slotkin JR, Cahill KS, Tharin SA, Shapiro EM. Cellular magnetic resonance imaging: nanometer and micrometer size particles for noninvasive cell localization. Neurotherapeutics 4, 2007; 428–33.
CrossRef
23. Kobayashi N, Noguchi H, Fujiwara T et al. Establishment of a highly differentiated immortalized adult human hepatocyte cell line by retroviral gene transfer. Transplant. Proc. 2000; 32, 2368–9.
CrossRef
24. Igaz P. Recent strategies to overcome the hyperacute rejection in pig to human xenotransplantation. Yale J. Biol. Med. 2001; 74, 329–40.
PMCid
25. Dai Y, Vaught TD, Boone J et al. Targeted disruption of the alpha1,3-galactosyltransferase gene in cloned pigs. Nat. Biotechnol. 2002; 20, 251–5.
CrossRef
26. Le Tissier P, Stoye JP, Takeuchi Y, Patience C, Weiss RA. Two sets of human-tropic pig retrovirus. Nature 1997; 389, 681–2.
CrossRef
27. Fabrega AJ, Bommineni VR, Blanchard J et al. Amelioration of analbuminemia by transplantation of allogeneic hepatocytes in tolerized rats. Transplantation 1995; 59, 1362–4.
PMid
28. Overturf K, Al-Dhalimy M, Tanguay R et al. Hepatocytes corrected by gene therapy are selected in vivo in a murine model of hereditary tyrosinaemia type I. Nat. Genet. 1996; 12, 266–73.
CrossRef
29. Ding J, Yannam GR, Roy-Chowdhury N et al. Spontaneous hepatic repopulation in transgenic mice expressing mutant human alpha1-antitrypsin by wild-type donor hepatocytes. J. Clin. Invest. 2011; 121, 1930–4.
CrossRef
30. Guha C, Sharma A, Gupta S et al. Amelioration of radiation-induced liver damage in partially hepatectomized rats by hepatocyte transplantation. Cancer Res. 1999; 59, 5871–4.
PMid
31. Zhou H, Dong X, Kabarriti R et al. Single liver lobe repopulation with wildtype hepatocytes using regional hepatic irradiation cures jaundice in Gunn rats. PLoS One 2012; 7, e46775.
CrossRef
32. Oertel M, Menthena A, Dabeva MD, Shafritz DA. Cell competition leads to a high level of normal liver reconstitution by transplanted fetal liver stem/progenitor cells. Gastroenterology 2006; 130, 507–20.
CrossRef
33. Haridass D, Yuan Q, Becker PD et al. Repopulation efficiencies of adult hepatocytes, fetal liver progenitor cells, and embryonic stem cell-derived hepatic cells in albumin-promoter-enhancer urokinase-type plasminogen activator mice. Am. J. Pathol. 2009; 175, 1483–92.
CrossRef
34. Grisham JW. A morphologic study of deoxyribonucleic acid synthesis and cell proliferation in regenerating rat liver; autoradiography with thymidine-H3. Cancer Res. 1962; 22, 842–9.
35. Fausto N & Campbell JS. The role of hepatocytes and oval cells in liver regeneration and repopulation. Mech. Dev. 2003; 120, 117–30.
CrossRef
36. Endo Y, Zhang M, Yamaji S, Cang Y. Genetic abolishment of hepatocyte proliferation activates hepatic stem cells. PLoS One 2012; 7, e31846.
CrossRef
37. Lemire JM, Shiojiri N, Fausto N. Oval cell proliferation and the origin of small hepatocytes in liver injury induced by D-galactosamine. Am. J. Pathol. 1991; 139, 535–52.
38. Shin S, Upadhyay N, Greenbaum LE, Kaestner KH. Ablation of Foxl1-Cre-labeled hepatic progenitor cells and their descendants impairs recovery of mice from liver injury. Gastroenterology 2015; 148, 192–202, e3.
CrossRef
39. Kuramitsu K, Sverdlov DY, Liu SB et al. Failure of fibrotic liver regeneration in mice is linked to a severe fibrogenic response driven by hepatic progenitor cell activation. Am. J. Pathol. 2013; 183, 182–94.
CrossRef
40. Gaia S, Smedile A, Omedè P et al. Feasibility and safety of G-CSF administration to induce bone marrow-derived cells mobilization in patients with end stage liver disease. J. Hepatol. 2006; 45, 13–9.
CrossRef
41. Pi L, Oh SH, Shupe T, Petersen BE. Role of connective tissue growth factor in oval cell response during liver regeneration after 2-AAF/PHx in rats. Gastroenterology 2005; 128, 2077–88.
CrossRef
42. Evans MJ & Kaufman MH. Establishment in culture of pluripotential cells from mouse embryos. Nature 1981; 292, 154–6.
CrossRef
43. Takayama K, Inamura M, Kawabata K et al. Generation of metabolically functioning hepatocytes from human pluripotent stem cells by FOXA2 and HNF1alpha transduction. J. Hepatol. 2012; 57, 628–36.
CrossRef
44. Woo DH, Kim SK, Lim HJ et al. Direct and indirect contribution of human embryonic stem cell-derived hepatocyte-like cells to liver repair in mice. Gastroenterology 2012; 142, 602–11.
CrossRef
45. Theise ND, Badve S, Saxena R et al. Derivation of hepatocytes from bone marrow cells in mice after radiation-induced myeloablation. Hepatology 2000; 31, 235–40.
CrossRef
46. Si-Tayeb K, Noto FK, Nagaoka M et al. Highly efficient generation of human hepatocyte-like cells from induced pluripotent stem cells. Hepatology 2010; 51, 297–305.
CrossRef
47. Yusa K, Rashid ST, Strick-Marchand H et al. Targeted gene correction of alpha1-antitrypsin deficiency in induced pluripotent stem cells. Nature 2011; 478, 391–4.
CrossRef
48. Cayo MA, Cai J, DeLaForest A et al. JD induced pluripotent stem cell-derived hepatocytes faithfully recapitulate the pathophysiology of familial hypercholesterolemia. Hepatology 2012; 56, 2163–71.
CrossRef
49. Rashid ST Corbineau S, Hannan N et al. Modeling inherited metabolic disorders of the liver using human induced pluripotent stem cells. J. Clin. Invest. 2010; 120, 3127–36.
CrossRef
50. Yu B, He ZY, You P et al. Reprogramming fibroblasts into bipotential hepatic stem cells by defined factors. Cell Stem Cell 2013; 13, 328–40.
CrossRef
51. Du Y, Wang J, Jia J et al. Human hepatocytes with drug metabolic function induced from fibroblasts by lineage reprogramming. Cell Stem Cell 2014; 14, 394–403.
CrossRef
52. Liu H, Kim Y, Sharkis S, Marchionni L, Jang YY. In vivo liver regeneration potential of human induced pluripotent stem cells from diverse origins. Sci. Transl. Med. 2011; 3, 82ra39.
CrossRef
53. Zhu S, Rezvani M, Harbell J et al. Mouse liver repopulation with hepatocytes generated from human fibroblasts. Nature 2014; 508, 93–7.
CrossRef
54. Russo FP, Alison MR, Bigger BW et al. The bone marrow functionally contributes to liver fibrosis. Gastroenterology 2006; 130, 1807–21.
CrossRef
55. Duffield JS, Forbes SJ, Constandinou CM et al. Selective depletion of macrophages reveals distinct, opposing roles during liver injury and repair. J. Clin. Invest. 2005; 115, 56–65.
CrossRef
56. Forbes SJ, Russo FP, Rey V et al. A significant proportion of myofibroblasts are of bone marrow origin in human liver fibrosis. Gastroenterology 2004; 126, 955–63.
CrossRef
57. Sakaida I, Terai S, Yamamoto N et al. Transplantation of bone marrow cells reduces CCl4-induced liver fibrosis in mice. Hepatology 2004; 40, 1304–11.
CrossRef
58. Yannaki E, Athanasiou E, Xagorari A et al. G-CSF-primed hematopoietic stem cells or G-CSF per se accelerate recovery and improve survival after liver injury, predominantly by promoting endogenous repair programs. Exp. Hematol. 2005; 33, 108–19.
CrossRef
59. Quintanilha LF, Mannheimer EG, Carvalho AB et al. Bone marrow cell transplant does not prevent or reverse murine liver cirrhosis. Cell Transplant. 2008; 17, 943–53.
CrossRef
60. Thomas JA, Pope C, Wojtacha D et al. Macrophage therapy for murine liver fibrosis recruits host effector cells improving fibrosis, regeneration, and function. Hepatology 2011; 53, 2003–15.
CrossRef
61. Wynn TA & Barron L. Macrophages: master regulators of inflammation and fibrosis. Semin. Liver Dis. 2010; 30, 245–57.
CrossRef
62. Ruhnke M, Ungefroren H, Nussler A et al. Differentiation of in vitro-modified human peripheral blood monocytes into hepatocyte-like and pancreatic islet-like cells. Gastroenterology 2005; 128, 1774–86.
CrossRef
63. Ramachandran P, Pellicoro A, Vernon MA et al. Differential Ly-6C expression identifies the recruited macrophage phenotype, which orchestrates the regression of murine liver fibrosis. Proc. Natl Acad. Sci. USA 2012; 109, E3186–95.
CrossRef
64. Kim JK, Park YN, Kim JS et al. Autologous bone marrow infusion activates the progenitor cell compartment in patients with advanced liver cirrhosis. Cell Transplant. 2010; 19, 1237–46.
CrossRef
65. Lyra AC, Soares MB, da Silva LF et al. Feasibility and safety of autologous bone marrow mononuclear cell transplantation in patients with advanced chronic liver disease. World J. Gastroenterol. 2007; 13, 1067–73.
CrossRef
66. Saito T, Okumoto K, Haga H et al. Potential therapeutic application of intravenous autologous bone marrow infusion in patients with alcoholic liver cirrhosis. Stem Cells Dev. 2011; 20, 1503–10.
CrossRef
67. Terai S, Ishikawa T, Omori K et al. Improved liver function in patients with liver cirrhosis after autologous bone marrow cell infusion therapy. Stem Cells 2006; 24, 2292–8.
CrossRef
68. Lyra AC, Soares MB, da Silva LF et al. Infusion of autologous bone marrow mononuclear cells through hepatic artery results in a short-term improvement of liver function in patients with chronic liver disease: a pilot randomized controlled study. Eur. J. Gastroenterol. Hepatol. 2010; 22, 33–42.
CrossRef
69. Spahr L, Chalandon Y, Terraz S et al. Autologous bone marrow mononuclear cell transplantation in patients with decompensated alcoholic liver disease: a randomized controlled trial. PLoS One 2013; 8, e53719.
CrossRef
70. Augello A, Kurth TB, De Bari C. Mesenchymal stem cells: a perspective from in vitro cultures to in vivo migration and niches. Eur. Cell Mater. 2010; 20, 121–33.
71. Tanimoto H, Terai S, Taro T et al. Improvement of liver fibrosis by infusion of cultured cells derived from human bone marrow. Cell Tissue Res. 2013; 354, 717–28.
CrossRef
72. Iwamoto T, Terai S, Hisanaga T et al. Bone-marrow-derived cells cultured in serum-free medium reduce liver fibrosis and improve liver function in carbon-tetrachloride-treated cirrhotic mice. Cell Tissue Res. 2013; 351, 487–95.
CrossRef
73. Miryounesi M, Piryaei A, Pournasr B, Aghdami N, Baharvand H. Repeated versus single transplantation of mesenchymal stem cells in carbon tetrachloride-induced liver injury in mice. Cell Biol. Intl 2013; 37, 340–7.
CrossRef
74. Mohamadnejad M, Alimoghaddam K, Mohyeddin-Bonab M et al. Phase 1 trial of autologous bone marrow mesenchymal stem cell transplantation in patients with decompensated liver cirrhosis. Arch. Iran. Med. 2007; 10, 459–66.
75. Kharaziha P, Hellström PM, Noorinayer B et al. Improvement of liver function in liver cirrhosis patients after autologous mesenchymal stem cell injection: a phase I-II clinical trial. Eur. J. Gastroenterol. Hepatol. 2009; 21, 1199–205.
CrossRef
76. Amer ME, El-Sayed SZ, El-Kheir WA et al. Clinical and laboratory evaluation of patients with end-stage liver cell failure injected with bone marrow-derived hepatocyte-like cells. Eur. J. Gastroenterol. Hepatol. 2011; 23, 936–41.
CrossRef
77. Peng L, Xie DY, Lin BL et al. Autologous bone marrow mesenchymal stem cell transplantation in liver failure patients caused by hepatitis B: short-term and long-term outcomes. Hepatology 2011; 54, 820–8.
CrossRef
78. Zhang Z, Lin H, Shi M et al. Human umbilical cord mesenchymal stem cells improve liver function and ascites in decompensated liver cirrhosis patients. J. Gastroenterol. Hepatol. 2012; 27 (Suppl 2), 112–20.
CrossRef
79. El-Ansary M, Abdel-Aziz I, Mogawer S et al. Phase II trial: undifferentiated versus differentiated autologous mesenchymal stem cells transplantation in Egyptian patients with HCV induced liver cirrhosis. Stem Cell Rev. 2012; 8, 972–81.
CrossRef
80. Shi M, Zhang Z, Xu R et al. Human mesenchymal stem cell transfusion is safe and improves liver function in acute-on-chronic liver failure patients. Stem Cells Transl. Med. 2012; 1, 725–31.
CrossRef
81. Jang YO, Kim YJ, Baik SK et al. Histological improvement following administration of autologous bone marrow-derived mesenchymal stem cells for alcoholic cirrhosis: a pilot study. Liver Intl 2014; 34, 33–41.
CrossRef
82. Kantarcioglu M, Demirci H, Avcu F, et al. Efficacy of autologous mesenchymal stem cell transplantation in patients with liver cirrhosis. Turk. J. Gastroenterol. 2015; 26, 244–50.
CrossRef
83. Salama H, Zekri AR, Medhat E et al. Peripheral vein infusion of autologous mesenchymal stem cells in Egyptian HCV-positive patients with end-stage liver disease. Stem Cell Res. Ther. 2014; 5, 70.
CrossRef
84. Lee JH, Lee KH, Kim MH et al. Possibility of undifferentiated human thigh adipose stem cells differentiating into functional hepatocytes. Arch. Plast. Surg. 2012; 39, 593–9.
CrossRef
85. Seo MJ, Suh SY, Bae YC, Jung JS. Differentiation of human adipose stromal cells into hepatic lineage in vitro and in vivo. Biochem. Biophys. Res. Commun. 2005; 328, 258–64.
CrossRef
86. Ong SY, Dai H, Leong KW. Hepatic differentiation potential of commercially available human mesenchymal stem cells. Tissue Eng. 2006; 12, 3477–85.
CrossRef
87. Luk JM, Wang PP, Lee CK, Wang JH, Fan ST. Hepatic potential of bone marrow stromal cells: development of in vitro co-culture and intra-portal transplantation models. J. Immunol. Methods 2005; 305, 39–47.
CrossRef
88. Rabani V, Shahsavani M, Gharavi M et al. Mesenchymal stem cell infusion therapy in a carbon tetrachloride-induced liver fibrosis model affects matrix metalloproteinase expression. Cell Biol. Intl 2010; 34, 601–5.
CrossRef
89. Volarevic V, Arsenijevic N, Lukic ML, Stojkovic M. Concise review: Mesenchymal stem cell treatment of the complications of diabetes mellitus. Stem Cells 2011; 29, 5–10.
CrossRef
90. Parekkadan B, van Poll D, Suganuma K et al. Mesenchymal stem cell-derived molecules reverse fulminant hepatic failure. PLoS One 2007; 2, e941.
CrossRef
91. Zhu X, He B, Zhou X, Ren J. Effects of transplanted bone-marrow-derived mesenchymal stem cells in animal models of acute hepatitis. Cell Tissue Res. 2013; 351, 477–86.
CrossRef
92. Xagorari A, Siotou E, Yiangou M et al. Protective effect of mesenchymal stem cell-conditioned medium on hepatic cell apoptosis after acute liver injury. Intl J. Clin. Exp. Pathol. 2013; 6, 831–40.
CrossRef
93. Fang B, Shi M, Liao L et al. Systemic infusion of FLK1(+) mesenchymal stem cells ameliorate carbon tetrachloride-induced liver fibrosis in mice. Transplantation 2004; 78, 83–8.
CrossRef
94. Chang YJ, Liu JW, Lin PC et al. Mesenchymal stem cells facilitate recovery from chemically induced liver damage and decrease liver fibrosis. Life Sci. 2009; 85, 517–25.
CrossRef
95. van Poll D, Parekkadan B, Cho CH et al. Mesenchymal stem cell-derived molecules directly modulate hepatocellular death and regeneration in vitro and in vivo. Hepatology 2008; 47, 1634–43.
CrossRef
96. di Bonzo LV, Ferrero I, Cravanzola C et al. Human mesenchymal stem cells as a two-edged sword in hepatic regenerative medicine: engraftment and hepatocyte differentiation versus profibrogenic potential. Gut 2008; 57, 223–31.
CrossRef
97. Meier RP, Muller YD, Morel P, Gonelle-Gispert C, Buhler LH. Transplantation of mesenchymal stem cells for the treatment of liver diseases, is there enough evidence? Stem Cell Res. 2013; 11, 1348–64.
CrossRef
98. am Esch 2nd JS, Knoefel WT, Klein M et al. Portal application of autologous CD133+ bone marrow cells to the liver: a novel concept to support hepatic regeneration. Stem Cells 2005; 23, 463–70.
99. Furst G, Schulte am Esch J, Poll LW et al. Portal vein embolization and autologous CD133+ bone marrow stem cells for liver regeneration: initial experience. Radiology 2007; 243, 171–9.
CrossRef
100. Gordon MY, Levicar N, Pai M et al. Characterization and clinical application of human CD34+ stem/progenitor cell populations mobilized into the blood by granulocyte colony-stimulating factor. Stem Cells 2006; 24, 1822–30.
CrossRef
101. Mohamadnejad M, Namiri M, Bagheri M et al. Phase 1 human trial of autologous bone marrow-hematopoietic stem cell transplantation in patients with decompensated cirrhosis. World J. Gastroenterol. 2007; 13, 3359–63.
CrossRef
102. Pai M, Zacharoulis D, Milicevic MN et al. Autologous infusion of expanded mobilized adult bone marrow-derived CD34+ cells into patients with alcoholic liver cirrhosis. Am. J. Gastroenterol. 2008; 103, 1952–8.
CrossRef
103. Gordon MY, Levicar N, Pai M et al. Characterization and clinical application of human CD34+ stem/progenitor cell populations mobilized into the blood by granulocyte colony-stimulating factor. Stem Cells 2006; 24, 1822–1830.
CrossRef
104. Levicar N, Pai M, Habib NA et al. Long-term clinical results of autologous infusion of mobilized adult bone marrow derived CD34+ cells in patients with chronic liver disease. Cell Prolif. 2008; 41 (Suppl.1), 115–25.
CrossRef
105. am Esch JS, Schmelzle M, Fürst G et al. Infusion of CD133+ bone marrow-derived stem cells after selective portal vein embolization enhances functional hepatic reserves after extended right hepatectomy: a retrospective single-center study. Ann. Surg. 2012; 255, 79–85.
CrossRef
106. Nikeghbalian S, Pournasr B, Aghdami N et al. Autologous transplantation of bone marrow-derived mononuclear and CD133(+) cells in patients with decompensated cirrhosis. Arch. Iran. Med. 2011; 14, 12–7.
107. Salama H, Zekri AR, Bahnassy AA et al. Autologous CD34+ and CD133+ stem cells transplantation in patients with end stage liver disease. World J. Gastroenterol. 2010; 16, 5297–305.
CrossRef
108. Salama H, Zekri AR, Zern M et al. Autologous hematopoietic stem cell transplantation in 48 patients with end-stage chronic liver diseases. Cell Transplant. 2010; 19, 1475–86.
CrossRef
109. Zekri AR Salama H, Medhat E et al. The impact of repeated autologous infusion of haematopoietic stem cells in patients with liver insufficiency. Stem Cell Res. Ther. 2015; 6, 118.
CrossRef
110. Salama H, Zekri AR, Ahmed R et al. Assessment of health-related quality of life in patients receiving stem cell therapy for end-stage liver disease: an Egyptian study. Stem Cell Res. Ther. 2012; 3, 49.
CrossRef
111. Lagasse E, Connors H, Al-Dhalimy M et al. Purified hematopoietic stem cells can differentiate into hepatocytes in vivo. Nat. Med. 2000; 6, 1229–34.
CrossRef
112. Orlic D, Kajstura J, Chimenti S et al. Bone marrow cells regenerate infarcted myocardium. Nature 2001; 410, 701–5.
CrossRef
113. Park KI, Teng YD, Snyder EY. The injured brain interacts reciprocally with neural stem cells supported by scaffolds to reconstitute lost tissue. Nat. Biotechnol. 2002; 20, 1111–7.
CrossRef
114. Hess D, Li L, Martin M et al. Bone marrow-derived stem cells initiate pancreatic regeneration. Nat. Biotechnol. 2003; 21, 763–70.
CrossRef
115. Alison MR, Poulsom R, Jeffery R et al. Hepatocytes from non-hepatic adult stem cells. Nature 2000; 406, 257.
CrossRef
116. Menthena A, Deb N, Oertel M et al. Bone marrow progenitors are not the source of expanding oval cells in injured liver. Stem Cells 2004; 22, 1049–61.
CrossRef
117. Jang YY, Collector MI, Baylin SB, Diehl AM, Sharkis SJ. Hematopoietic stem cells convert into liver cells within days without fusion. Nat. Cell Biol. 2004; 6, 532–9.
CrossRef
118. Vassilopoulos G, Wang PR, Russell DW. Transplanted bone marrow regenerates liver by cell fusion. Nature 2003; 422, 901–4.
CrossRef
119. Wang X, Willenbring H, Akkari Y et al. Cell fusion is the principal source of bone-marrow-derived hepatocytes. Nature 2003; 422, 897–901.
CrossRef
120. Muraca M, Ferraresso C, Vilei MT et al. Liver repopulation with bone marrow derived cells improves the metabolic disorder in the Gunn rat. Gut 2007; 56, 1725–35.
CrossRef
121. Vig P, Russo FP, Edwards RJ et al. The sources of parenchymal regeneration after chronic hepatocellular liver injury in mice. Hepatology 2006; 43, 316–24.
CrossRef
122. Nishino T, Hisha H, Nishino N, Adachi M, Ikehara S. Hepatocyte growth factor as a hematopoietic regulator. Blood 1995; 85, 3093–100.
123. Selzner N, Selzner M, Odermatt B et al. ICAM-1 triggers liver regeneration through leukocyte recruitment and Kupffer cell-dependent release of TNF-alpha/IL-6 in mice. Gastroenterology 2003; 124, 692–700.
CrossRef
124. Fausto N, Campbell JS, Riehle KJ. Liver regeneration. Hepatology 2006; 43, S45–53.
CrossRef
125. Higashiyama R, Inagaki Y, Hong YY et al. Bone marrow-derived cells express matrix metalloproteinases and contribute to regression of liver fibrosis in mice. Hepatology 2007; 45, 213–22.
CrossRef
126. de Oliveira SA, de Freitas Souza BS, Sá Barreto EP et al. Reduction of galectin-3 expression and liver fibrosis after cell therapy in a mouse model of cirrhosis. Cytotherapy 2012; 14, 339–49.
CrossRef
127. Jitraruch, S, Dhawan A, Hughes RD et al. Alginate microencapsulated hepatocytes optimised for transplantation in acute liver failure. PLoS One 9, 2014; e113609.
CrossRef
128. Nagata H, Ito M, Shirota C et al. Route of hepatocyte delivery affects hepatocyte engraftment in the spleen. Transplantation 2003; 76, 732–4.
CrossRef
129. Couto BG, Goldenberg RC, da Fonseca LM et al. Bone marrow mononuclear cell therapy for patients with cirrhosis: a Phase 1 study. Liver Intl 2011; 31, 391–400.
CrossRef
130. Slehria S, Rajvanshi P, Ito Y et al. Hepatic sinusoidal vasodilators improve transplanted cell engraftment and ameliorate microcirculatory perturbations in the liver. Hepatology 2002; 35, 1320–8.
CrossRef
131. Bahde R, Kapoor S, Bandi S et al. Directly acting drugs prostacyclin or nitroglycerine and endothelin receptor blocker bosentan improve cell engraftment in rodent liver. Hepatology 2013; 57, 320–30.
CrossRef
132. Viswanathan P, Kapoor S, Kumaran V, Joseph B, Gupta S. Etanercept blocks inflammatory responses orchestrated by TNF-alpha to promote transplanted cell engraftment and proliferation in rat liver. Hepatology 2014; 60, 1378–88.
CrossRef