The role of biopreservation in cell and gene therapy bioprocessing
Cell Gene Therapy Insights 2017; 3(5), 335-344.
10.18609/cgti.2017.037
Cell and gene-based therapies represent a novel therapeutic modality that has the potential to provide a treatment option for a range of medical conditions. There are, however, numerous processing and manufacturing challenges that must be addressed before such therapies are considered commercially and clinically viable. A significant challenge associated with the manufacture of such therapies is ensuring cell quality and the product’s critical quality attributes are retained throughout the entire bioprocess. Biopreservation is an important aspect of cell and gene-based therapy bioprocessing, which enables the development of cell banks. It increases process flexibility by providing a shelf-life to the product, enables the storage of intermediates and provides breakpoints within the process. In this article, we summarize the advances and challenges associated with biopreservation of cell and gene therapies.
Cell and gene-based therapies (CGTs) represent a novel therapeutic modality that have the potential to address the significant medical challenges associated with chronic, age-related clinical indications and provide a treatment option for a range of unmet medical needs. There are, however, numerous processing and manufacturing challenges that must be addressed before such therapies are considered commercially and clinically viable. A significant challenge associated with the manufacture of such therapies is ensuring cell quality and that the product’s critical quality attributes (CQAs) [1] are retained throughout the entire bioprocess, from initial cell isolation through to delivery and administration to a patient. Unlike traditional biopharmaceutical manufacture, manufacturing paradigms for CGTs may involve an autologous (patient-specific), allogeneic (universal donor) or haplobank approach.
An important aspect of all CGT bioprocessing is biopreservation. This enables the creation of cell banks (master and working cell banks for allogeneic product manufacture or storage banks for patient specific material such as cord blood). This can uncouple the expansion and/or manipulation of cells from the clinic, creating product shelf-life and also facilitates the transport/storage of any product intermediates (e.g., newly isolated cells or even viral vectors)(Figure 1)[2].
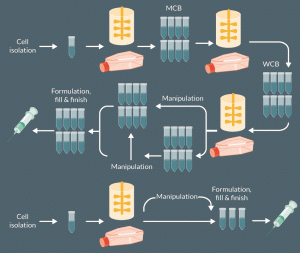
Figure 1: Simplified overview of a cell therapy manufacturing process showing when biopreservation (shown by vials) may be applied. The manipulation indicated would vary from therapy to therapy or may not be needed but could for example include differentiation of iPSC cells to cells of therapeutic interest, genetic engineering of T-cells for CAR T cells production. For the creation of cell banks, be it master cell banks for allogeneic products or single vials of patient specific material stored for later use, cryopreservation is the most commonly used option. However, hypothermic storage at ambient or refrigerated temperatures may enable short shelf lives of some ‘fresh’ products to be achieved.
Indeed, where the process necessitates transfer of cellular material from the clinic to an off-site facility for processing and/or expansion, biopreservation measures will be critical to product quality and success. This places particular emphasis on the appropriate selection of biopreservation methods, with a need to demonstrate that they do not adversely impact the product’s CQAs. Moreover, the biopreservation methodology needs to align with the business and distribution model to ensure commercial success and should occur during early stages of development given the integral role biopreservation plays in the logistical and manufacturing bioprocessing considerations [3].Biopreservation also determines the level of at-clinic processing, that is, will the cells need to be thawed prior to administration or are they preserved and subsequently delivered at ambient temperature. Thus, in many CGT processes, it is likely that biological material will require at least one biopreservation step, if not more (Figure 1). This will be exacerbated for gene therapy products where other biological material (such as viral vectors) are also likely to undergo an independent preservation process prior to their addition to the cellular material.
Types of biopreservation
Biopreservation is often used synonymously with cryopreservation. However, whilst a review of the regulatory submissions for mesenchymal stem cell therapies to the FDA in 2012 reveals that freezing is certainly a key biopreservation method, with more than 80% of submissions involving a frozen product [4],it is not the only biopreservation method that is being considered for commercial and clinical use. Indeed, given the need to retain the product CQAs and that multiple different manufacturing/distribution models are likely to be employed for CGTs (e.g., autologous, allogeneic and haplobank), it has become increasingly important to consider other types of biopreservation for the storage and transportation of biological material. This includes hypothermic preservation (i.e., ‘cell pausing’) at either ambient [5-7] or refrigerated chilled temperatures [8,9].Ultimately, the decision as to which biopreservation method is most appropriate will need to take into account which method has the least effect on the product’s CQAs whilst remaining both commercially, clinically and logistically viable.
Cryopreservation
Freezing is the most common biopreservation method employed in CGT processes [2],with the first successful cryopreservation taking place in 1949 with spermatozoa in glycerol [10]. Standard cryopreservation practise of cellular material usually involves a two-step controlled rate freezing (CRF) process: (1) the controlled reduction of the temperature of the cellular material to -80˚C at a rate of 1 ˚C /min, followed by, (2) reducing the temperature even further to less than -150˚C, usually by placing into liquid nitrogen, its vapour phase for long-term storage. This method of CRF has been demonstrated for both millilitre cryovials and in bags of large volumes (>100 mL) for both adult stem cells [11] and other mammalian cells [12,13]. It has also been successfully employed for the human fibroblast-derived dermal substitute Dermagraft (Advanced Biohealing, USA) [14] in addition to the human mesenchymal stem cell (hMSC) product, Prochymal, which has received conditional approval in New Zealand and Canada, and is available in the United States under an Expanded Access Programme for treatment of acute graft versus host disease in children [15,16]. In the Health Canada summary basis of decision, Prochymal’s shelf-life is identified as 2 years at less than or equal to -135˚C [16].
Cryopreservation usually enables high cell recovery, with more than 90% cell viability immediately post-thaw. However, this is cell and process-dependent and delayed onset apoptosis can be caused [17], which is not detected immediately post-thaw by assays such as trypan blue exclusion. Indeed, there have been studies which, for example, have demonstrated that human embryonic stem cells are sensitive to cryopreservation with a relatively low recovery post-thaw, in addition to changes in cell phenotype, resulting in poor outcomes for cell functionality [18,19]. Moreover, whilst some studies have demonstrated an adverse effect on hMSC functionality with respect to T-cell suppression, response to IFN-γ, lower production of anti-inflammatory mediators and impaired blood regulatory properties immediately post-thaw for hMSCs compared with actively proliferating hMSCs [20,21], yet others have demonstrated that cryopreservation does not adversely affect the viability, immunomodulatory properties or differentiation capacity [22]. For example, Cruz et al. reported equivalency between cryopreserved and non-cryopreserved hMSCs [23]. François et al, one of the groups who reported that hMSCs were adversely impacted by cryopreservation did note that thawed hMSCs recovered their immunomodulatory functionality 24 hours post-culture [20]suggesting that a cell-recovery process may need to be incorporated post-thaw to retain viability and, more importantly, ensure functionality of the cryopreserved cells. If indeed this is necessary for the product to retain its CQAs, this increases process complexity and costs, with a particular consideration as to where the cell-recovery process step is conducted. Moreover, the differences between the aforementioned studies could also potentially be explained by differences in the cell handling, cryopreservation medium formulations, freezing protocols and thawing procedures. That the thawing process is often badly, if at all, controlled and that this may be responsible for some of the damage perceived to be caused by cryopreservation is now starting to be recognised by the community. For example, at ISCT 2017, one report highlighted the interplay between cooling rate and thawing rate for T cell recovery post-thaw [24]. To address this, rapid and specialised thawing devices are being developed such as the ThawSTAR® from Medcision and VIA Thaw by Asymptote.
As well as CRF, vitrification can also be used to cryopreserve cells. This involves the rapid cooling of the cellular material, thereby avoiding formation of both extra- and intra-cellular ice crystals [25] and generating amorphous ice. Although vitrification has been employed successfully for multiple cell types, in particular, colonies of human embryonic stem cells, where vitrification has resulted in high levels of viability and retention of functionality post-thaw [26-28]. However, this method uses significantly higher levels of cryoprotective agent (CPA) than in CRF to prevent ice crystallisation, and enable vitrification at a higher glass transition temperature and an achievable cooling rate. Also, although efforts are ongoing to generate larger scale vitrification methods, the high cooling rates needed mean that only small volumes (typically 1-20 µl) may be vitrified at once [3], making this a poor option for the freezing of cell therapies currently.
Cryopreservation challenges
Although cryopreservation is currently considered to be the industry standard for CGT biopreservation, there are numerous challenges and considerations. Primarily, it is critical to understand the implications of cryopreservation on the product’s CQAs. This requires in-depth understanding about the product’s biological attributes, mechanism of action and safety profile. There are also concerns with the use of liquid nitrogen in a GMP environment, where it may compromise clean room air quality given the potential for contamination with microorganisms, particulates and debris during transport and storage [29-31]. To use liquid nitrogen in a clean GMP facility would require the use of validated sterilization and/or filtration methods [31,32]. This can, however, be overcome by omitting the use of liquid nitrogen and using an electrically-powered Stirling cryocooler, which has been used for multiple human cell types, including human embryonic stem cells and alginate-encapsulated liver cells [31].
The most significant concern with cryopreservation is the use of CPAs, in particular the intracellular CPA, dimethyl sulphoxide (DMSO). DMSO, the most commonly used CPA, is classified by the FDA as a class 3 solvent [33] and is considered to be toxic to both cells and patients at room temperature. It is recommended that patients do not receive more than 1g/kg body weight/day of DMSO, with a maximum permissible limit of 50 mg/day [34,35]. Such concerns and restrictions have been put in place due to the toxicology issues associated with DMSO [36]. This concern is exacerbated with vitrification, given the need to significantly increase the CPA concentrations to avoid ice crystal formation. Although DMSO is still the most commonly used CPA, alternatives are being considered for the cryopreservation of mammalian cells that pose a lower toxicity risk including trehalose [37] and sucrose [38]. These CPAs provide a similar level of protection to cellular material during freezing but the challenge with these CPAs is their inability to penetrate the cell. The delivery of these CPAs into mammalian cells requires an additional process step, such as electroporation or permeabilization [37,39,40]. Such procedures alone can lead to cell death and destruction.
Hypothermic storage
Whilst it is generally recognised and accepted that for long-term storage (months/years), cryopreservation remains the most cost-effective and scientifically robust method, there are genuine concerns about the use of cryopreservation for short-term storage and transport due to the potential for ice crystal formation and potentially toxic CPA concentrations. A potential alternative biopreservation method that is amenable for short-term requirements (e.g., days) is hypothermic storage. This includes chilled preservation, a process where the temperature is reduced to 2–8˚C and ambient preservation (room temperature). Refrigerated preservation is currently used for the transfusion of red blood cells for the treatment of severe anaemia. Red blood cells can be stored at refrigeration temperature for up to 42 days within an additive solution, where they can then be transported to the site of administration upon request [41]. Moreover, refrigeration temperatures have also been used for the storage and transportation or whole organs prior to transplantation [42,43].
Much of the research and development activity for chilled preservation relates to storage and transportation of whole organs and red blood cells. However, with the increasing promise of CGTs, there has been renewed interest in short-term chilled preservation for CGT cell candidates, in particular, stem cells. The commercial hypothermic preservation medium solution HypoThermosol® Free Radical Solution (HTS-FRS) (BioLife Solutions, USA) has been used for the successful storage and preservation of a range of cell types including hMSCs [44] and differentiated human neural stem cells [45]. In the case of hMSCs, the cells retained greater than 85% viability after 96 hours when stored at 4˚C [44], and for the differentiated human neural stem cells, the cells were seeded onto cellular collagen constructs and placed into a range of different medium compositions for storage at 4˚C for 48 h. The cells which were preserved in the HTS-FRS medium composition demonstrated the least cell death (<10%), even lower than cells which were preserved using DMSO under cryogenic conditions [45].Indeed, recently, HTS-FRS was used for TiGenix’s Phase 1 clinical trial where expanded allogeneic adipose-derived stem cells stored in HTS-FRS were administered to patients [46].
Although chilled preservation will not be used as a long-term biopreservation method, it has demonstrated significant potential for its ability to suitably preserve cells at refrigerated temperatures for time periods accounting for transportation across countries. Transporting a CGT product between countries a significant distance apart can take up to 24 h, however this does not take into account transportation delays, customs checks and potential clinical complications. As such, the longer the cells can be shown to demonstrate the retention of their CQAs in the biopreservation formulation, the more flexibility this provides to the overall process [3]. The additional advantage of this type of preservation in comparison to cryopreservation is that much of the clinical infrastructure, logistics and supply chain aspects to enable chilled storage and transportation is already in place. As such, significant changes to current practice and facilities will unlikely be required.
Ambient temperature preservation simplifies the process even further compared to chilled preservation and does not require any additional infrastructure to maintain refrigerated or cryogenic temperatures, thereby reducing cost and process complexity. Unlike cryopreservation and, to a lesser extent, chilled preservation, the research with ambient temperature preservation is not as extensive and has only become an area of interest in light of the potential advantages this preservation method confers over the aforementioned techniques. The potential of this technique is demonstrated by Chen et al. (2013) who demonstrated that they were able to store hMSCs and mouse embryonic stem cells inside alginate hydrogels for 5 days in ambient conditions in an air-tight environment. Cell viability post-preservation was found to be 80% and 74% for the hMSCs and mouse embryonic stem cells, respectively [47]. Similarly, Swioklo et al., showed human adipose-derived stem cells could be encapsulated and stored at a range of temperatures, from 4–23°C, although 15°C was found to be the optimum [48]. The disadvantage to this method, however, is the need for a cell retrieval step which increases process complexity and will likely need to be undertaken at or near clinic. Ambient temperature cell preservation has also been reported for hair follicles which demonstrated adequate graft recovery post-preservation [49]. Additionally, Hunt et al., have reported the successful preservation of other mammalian cell types (CHO and HEK293 cells) at temperatures ranging between 6–24˚C for more than 3 days [6].
As with chilled preservation, ambient temperature preservation is a potential technique that can be used for pooling of cells during the process (for example post-harvest from culture devices awaiting downstream processing), or indeed, used for storage and transportation to site of clinical administration. However, it is critical that the acceptable temperature ranges and timeframes are identified and validated, with the validated conditions making allowances for potential processing and/or transport delays. There should also be consideration of effect of multiple preservation steps (if employed) during a process and the cumulative effect of ambient temperature preservation throughout the process. Greater product and process understanding will facilitate the consideration of multiple processing options and increase overall process flexibility.
Hypothermic preservation challenges
There are two key challenges for chilled preservation. The first has been alluded to previously and relates to the maximum time-period it can be effectively used, and importantly, validated, as a biopreservation method. Although there has been promising research data that suggest chilled preservation in conjunction with specifically designed hypothermic medium preservation solutions can retain cell viability and functionality across multiple days, there is a greater burden of evidence required for validation.
The other key challenge with chilled preservation, and exacerbated for ambient preservation (discussed later), is the fact that chilled preservation only slows and does not arrest cellular functions, activity and metabolism. This results in the potential build-up of deleterious metabolites and, perhaps more critically, the depletion of important substrates required for cell maintenance [50,51].It should also be noted that although chilled preservation does not result in cellular damage arising from ice crystal formation or suffer from CPA toxicity issues experienced with cryopreservation, there are concerns about chilled preservation resulting in cold-induced cell damage [52].This risk of cell damage is intrinsically linked to the preservation time, with a greater risk of cell damage and decreased viability with longer preservation times [3]. There are also concerns with chilled preservation that once rewarmed to the appropriate administration temperature, the increased production of free radicals and other deleterious metabolites may result in delayed-onset cell death, which may not be noticeable immediately but take hours or days to become apparent [53].
A key challenge with ambient temperature preservation is demonstrating the utility and efficacy of such a preservation at industrial scales and practical terms. Given the relative infancy of this area of research, it is expected that further studies will be conducted outlining the promise and potential of this technique. However, at present, there is little that has been conducted with relevant cell types with a focus on CGT manufacture and bioprocessing to be able to make a strong scientific case for ambient temperature preservation. However, should it be scientifically feasible, the advantages of ambient temperature preservation are substantial and would significantly reduce process, storage and transportation costs.
Translational insight
Biopreservation of biological material for CGTs is often considered too late in process development or not given due attention because of what is often perceived as other, ‘more important’ development activities. However, for effective CGT process development, given its pivotal role in process development, storage, transportation and overall business model, it is critical to identify early in process development which biopreservation technique(s) (cryo-, chilled, or ambient preservation) is the most appropriate for the CGT. Cryopreservation will remain the only feasible biopreservation technique for long-term storage (months/years); however, both chilled and ambient preservation methods provide additional process flexibility, particularly with respect to interprocess pooling/storage and transportation across manufacturing and clinical facilities. As with any CGT process, it is unlikely that a universal biopreservation approach will be appropriate for all cases and ultimately the process needs to be determined after thorough development activity to identify effects of biopreservation on the product’s CQAs and the implications of the technique on the distribution and commercial models.
References
1. Brandenberger R, Burger SR, Campbell A et al. Cell therapy bioprocessing. Bioprocess International. 2011: 9 (30–37).
Website
2. Coopman K and Medcalf N. From production to patient: challenges and approaches for delivering cell therapies, in StemBook The Stem Cell Research Community 2014;
Crossref
3. Jesson HE, Robinson NJ, Medcalf N, Coopman K. Storage and Delivery of Stem Cells for Cellular Therapies in Cabral et al. (Eds) Stem Cell Manufacturing 2016; 233–64
4. Mendicino M et al. MSC-based product characterization for clinical trials: an FDA perspective. Cell Stem Cell 2014; 14(2): 141–5.
Crossref
5. Robinson NJ, Picken A, and Coopman K. Low temperature cell pausing: an alternative short-term preservation method for use in cell therapies including stem cell applications. Biotechnol Lett. 2014; 36(2): 201–9.
Crossref
6. Hunt L et al. Low-temperature pausing of cultivated mammalian cells. Biotechnol Bioeng. 2005; 89(2): 157–63.
Crossref
7. Eidet JR et al. The impact of storage temperature on the morphology, viability, cell number and metabolism of cultured human conjunctival epithelium. Curr Eye Res, 2015; 40(1): 30–9.
Crossref
8. Matsumoto N et al. Successful liquid storage of peripheral blood stem cells at subzero non-freezing temperature. Bone Marrow Transplant 2002; 30(11): 777–84.
Crossref
9. Moce-Llivina L and Jofre J. A method to maintain mammalian cells for days alive at 4 degrees C. Cytotechnology 2004; 46(1): 57–61
Crossref
10. Polge C, Smith AU, Parkes AS. Revival of spermatozoa after vitrification and dehydration at low temperatures. Nature 1949; 164(4172): 666
Crossref
11. Sputtek A, Lioznov M, Kröger N, Rowe AW. Bioequivalence comparison of a new freezing bag (CryoMACS®) with the Cryocyte®) freezing bag for cryogenic storage of human hematopoietic progenitor cells. Cytotherapy 2011; 13(4): 481–9
Crossref
12. Heidemann R, Mered M, Wang DQ et al. A new seed-train expansion method for recombinant mammalian cell lines. Cytotechnology 2002; 38(1-3): 99–108
Crossref
13. Heidemann R, Lünse S, Tran D, Zhang C. Characterization of cell-banking parameters for the cryopreservation of mammalian cell lines in 100-mL cryobags. Biotechnol Prog. 2010; 26(4): 1154–63
Crossref
14. Mason C & Manzotti E. Regenerative medicine cell therapies: numbers of units manufactured and patients treated between 1988 and 2010. Regen Med. 2010; 5(3): 307–13
Crossref
15. Mesoblast Provides Update on Clinical Programs of Prochymal for Crohn’s Disease and Acute Graft Versus Host Disease 2014
Website
16. Health Canada. Summary Basis of Decision (SBD) for PROCHYMAL®. 2012
Website
17. Van Buskirk RG, Snyder KK, Baust JG et al. Cryopreservation: It’s not just about cell yield. Bioprocess International 2005; 3(4): 64–72
Website
18. Li Y, Tan JC, Li LS. Comparison of three methods for cryopreservation of human embryonic stem cells. Fertil Steril 2010; 93(3): 999–1005.
Crossref
19. Xu X et al. The roles of apoptotic pathways in the low recovery rate after cryopreservation of dissociated human embryonic stem cells. Biotechnol Prog 2010; 26(3): 827–37
Crossref
20. François M, Copland IB, Yuan S et al. Cryopreserved mesenchymal stromal cells display impaired immunosuppressive properties as a result of heat-shock response and impaired interferon-γ licensing. Cytotherapy 2012; 14(2): 147–152.
Crossref
21. Moll G, Alm JJ, Davies LC et al. Do Cryopreserved Mesenchymal Stromal Cells Display Impaired Immunomodulatory and Therapeutic Properties? Stem cells (Dayton, Ohio) 2014; 32(9): 2430–2442.
Crossref
22. Luetzkendorf J, Nerger K, Hering J et al. Cryopreservation does not alter main characteristics of Good Manufacturing Process-grade human multipotent mesenchymal stromal cells including immunomodulating potential and lack of malignant transformation. Cytotherapy 2015; 17(2): 186–98
Crossref
23. Cruz FF, Borg ZD , Goodwin M et al. Freshly thawed and continuously cultured human bone marrow-derived mesenchymal stromal cells comparably ameliorate allergic airways inflammation in immunocompetent mice. Stem Cells Transl Med 2015; 4(6): 615–24
Crossref
24. Baboo J et al. The important relationship between cooling and thawing rates on human T cell quality. Cytotherapy. 19(5): S29.
Crossref
25. Heo YS et al. “Universal” vitrification of cells by ultra-fast cooling. Technology, 2015. 3(1): 64-71.
Crossref
26. Beier AF, Schulz JC, and Zimmermann H. Cryopreservation with a twist – towards a sterile, serum-free surface-based vitrification of hESCs. Cryobiology 2013. 66(1): 8–16
Crossref
27. Hunt CJ & Timmons PM. Cryopreservation of human embryonic stem cell lines. Methods Mol Biol 2007. 368: 261–70.
Crossref
28. Li T, Zhou C, Liu C et al. Bulk vitrification of human embryonic stem cells. Hum Reprod 2008; 23(2): 358–64.
Crossref
29. Fountain D, Ralston M, Higgins N, et al. Liquid nitrogen freezers: a potential source of microbial contamination of hematopoietic stem cell components. Transfusion 1997; 37(6): 585–91.
Crossref
30. Bielanski A & Vajta G. Risk of contamination of germplasm during cryopreservation and cryobanking in IVF units. Hum Reprod 2009; 24(10): 2457–67.
Crossref
31. Massie I et al. GMP Cryopreservation of Large Volumes of Cells for Regenerative Medicine: Active Control of the Freezing Process. Tissue Engineering. Part C, Methods. 2014; 20(9): 693–702.
Crossref
32. Parmegiani L, Accorsi A, Cognigni GE, et al., Sterilization of liquid nitrogen with ultraviolet irradiation for safe vitrification of human oocytes or embryos. Fertil Steril 2010; 94(4): 1525–8.
Crossref
33. Center for Biologics Evaluation and Research (CBER). Guidance for Industry: Q3C – Tables and Lists, U.S. Food and Drug Administration (FDA) 2012
Website
34. International Conference on Harmonization (ICH). Impurities: Guideline for Residual Solvents Q3C(R6). ICH Harmonised Tripartite Guideline 2011
Website
35. Pomper GJ. Febrile, Allergic, and Nonimmune Transfusion Reactions in Rossi’s Principles of Transfusion Medicine. 2009; Wiley-Blackwell; 826–846.
Crossref
36. Brobyn RD. The human toxicology of dimethyl sulfoxide. Annals of the New York Academy of Sciences 1975; 243(1): 497–506.
Crossref
37. Sharp DMC, Picken A, Morris TJ, et al. Amphipathic polymer-mediated uptake of trehalose for dimethyl sulfoxide-free human cell cryopreservation()(). Cryobiology 2013; 67(3): 305–311.
Crossref
38. Hossain AM & Osuamkpe CO. Sole use of sucrose in human sperm cryopreservation. Arch Androl 2007; 53(2): 99–103.
Crossref
39. Eroglu A, Russo MJ, Bieganski R et al. Intracellular trehalose improves the survival of cryopreserved mammalian cells. Nat Biotechnol 2000; 18(2): 163–7.
Crossref
40. Zhou X, Yuan J, Liu J et al. Loading trehalose into red blood cells by electroporation and its application in freeze-drying. Cryo Letters 2010; 31(2): 147–56.
Website
41. Hess JR. Scientific problems in the regulation of red blood cell products. Transfusion 2012; 52(8): 1827–35.
Crossref
42. Collins GM, Bravo-Shugarman M, Terasaki PI. Kidney preservation for transportation. Initial perfusion and 30 hours’ ice storage. Lancet 1969; 2(7632): 1219–22.
Crossref
43. Lee CY & Mangino MJ. Preservation methods for kidney and liver. Organogenesis 2009; 5(3): 105–112.
Crossref
44. Ginis IB, Grinblat, Shirvan MH. Evaluation of bone marrow-derived mesenchymal stem cells after cryopreservation and hypothermic storage in clinically safe medium. Tissue Eng Part C Methods, 2012. 18(6): 453–63.
Crossref
45. Day AGE et al. Hypothermic and cryogenic preservation of artificial neural tissue made using differentiated CTX human neural stem cells in collagen gels. European Cells and Materials, 2015; 29(3): 44
Website
46. ClinicalTrials.gov. Intralymphatic eASC Administration in Healthy Volunteers. ClinicalTrials.gov Identifier: NCT01743222 2013
Website
47. Chen B et al. A novel alternative to cryopreservation for the short-term storage of stem cells for use in cell therapy using alginate encapsulation. Tissue Eng Part C Methods, 2013; 19(7): 568–76.
Crossref
48. Swioklo SA, Constantinescu A, Connon CJ. Alginate-Encapsulation for the Improved Hypothermic Preservation of Human Adipose-Derived Stem Cells. Stem Cells Transl Med, 2016; 5(3): 339–49.
Crossref
49. Parsley WM & Perez-Meza D. Review of Factors Affecting the Growth and Survival of Follicular Grafts. Journal of Cutaneous and Aesthetic Surgery 2010; 3(2): 69–75.
DOI
50. Reyes BA, Pendergast JS & Yamazaki S. Mammalian Peripheral Circadian Oscillators Are Temperature Compensated. Journal of Biological Rhythms 2008. 23(1): 95–98.
Crossref
51. Plesnila N, Müller E, Guretzki Set al., Effect of hypothermia on the volume of rat glial cells. The Journal of Physiology 2000. 523(Pt 1): 155–162.
Crossref
52. Baust JG, Gao D, Baust JM. Cryopreservation: an emerging paradigm change. Organogenesis 2009. 5(3): 90–96
Crossref
53. Baust JM. Properties of Cells and Tissues Influencing Preservation Outcome. Advances in Biopreservation 2006, CRC Press 63–87.
Crossref
Affiliations
Qasim A Rafiq1,2, Alvin W Nienow3, Christopher J Hewitt2 & Karen Coopman4
1 Advanced Centre for Biochemical Engineering,
Department of Biochemical
Engineering, University College London,
London, WC1E 6BT, United Kingdom
2 Aston Medical Research Institute, School
of Life and Health Sciences, Aston University,
Birmingham, B4 7ET, United
Kingdom
3 School of Chemical Engineering, University
of Birmingham, Edgbaston, Birmingham,
B15 2TT, United Kingdom
4 Centre for Biological Engineering,
Loughborough University, Leicestershire,
LE11 3TU, United Kingdom
*Author for Correspondence:
Dr Qasim Rafiq, Advanced Centre for
Biochemical Engineering, Department
of Biochemical Engineering, University
College London, Gower Street, London,
WC1E 6BT, United Kingdom
E-mail: q.rafiq@ucl.ac.uk
This work is licensed under a Creative Commons Attribution- NonCommercial – NoDerivatives 4.0 International License.